Nitrogen Annealing As a Sustainable Method for Interface Trap Passivation in 4H-SiC Mosfets
ECS Meeting Abstracts(2022)
摘要
Silicon Carbide (4H-SiC) has emerged as a leading wide band gap semiconductor for high-power, high-temperature applications1. 4H-SiC metal-oxide semiconductor-field-effect transistors (MOSFETs) have lower power dissipation compared to silicon, allowing for low-noise and high-efficiency all-electric vehicle drives, fast-charging stations, solar inverters, and more. While these devices provide substantial advancements for next-generation energy efficient power systems, 4H-SiC may also offer additional functionality in the form of integrated circuits (ICs) at high temperatures (>300 °C). Because of its high noise immunity and low static power consumption, lateral complementary-metal-oxide-semiconductor (CMOS) IC technology in 4H-SiC is desirable for large-scale integration2. This technology necessitates the use of both n- and p-channel MOSFETs that can operate at high temperatures. Despite the advances of 4H-SiC MOSFETs, the high density of interface states (Dit) at the 4H-SiC/SiO2 interface prevents reaching full potential resulting in high channel resistance and low mobility. Alternatives to nitric oxide (NO) annealing, the most common method adopted to reduce Dit in 4H-SiC3,4, are actively sought due to its toxicity and relatively expensive cost. Annealing in pure nitrogen (N2)5,6 at high temperatures (1400 °C-1600 °C) has been recently demonstrated promising results for 4H-SiC MOSFET processing. In this work, we report Dit measurements consistent with [6] and attempt to correlate the nitrogen areal densities of the near interfacial regions with the Dit for high temperature N2 annealing processes compared to NO. In our study, metal oxide semiconductor capacitors were fabricated on p- and n-type 4H-SiC epitaxial layers. Gate oxides were thermally grown at 1150 °C for 10 h in dry O2 resulting in a ~ 60 nm thick oxide layer. Selected samples are then annealed in flowing N2 at high temperatures (1400 °C, 1 h; 1450 °C, 1 h; and 1500 °C, 30 minutes or 1 h) or NO (1175 °C, 2 h). X-ray photoelectron spectroscopy (XPS), carried out after etching the oxide, indicates that the amount of nitrogen at the interface due to high temperature N2 annealing is ~ 4 × higher than NO annealed devices. Simultaneous high frequency (100 kHz)- low frequency CV was performed to extract interface trap densities (Dit) for each process and compared at room temperature (27 °C) with reference 1175 °C, 2 h NO annealed samples. The comparison reveals that, N2 annealing at 1500 °C for 30 minutes with a flow rate of 3 LPM results in Dit values comparable to NO annealing across the bandgap. Moreover, nitrogen annealing is more effective in reducing Dit near the valence band than NO annealing, while the opposite is true close to the conduction band-edge, consistent with previous reports [6] and observed in atomistic models of these interfaces using the density functional theory7. Nitrogen annealing also decreases the positive fixed charges at the interface of p-type 4H-SiC and SiO2, as evidenced from the flat band voltage comparison. The oxide breakdown voltage for the devices made with 1500 °C N2 annealing was similar to that of NO annealed devices. XPS analysis of the N2 annealed devices, their behavior under high temperature and bias, and their potential to substitute NO will be further discussed. The authors gratefully acknowledge the support from the National Renewable Energy Laboratory/ US Department of Energy sub-contract NREL-AHL-9-92362-01. References: 1 T. Kimoto and J.A. Cooper, Fundamentals of Silicon Carbide Technology: Growth, Characterization, Devices and Applications (John Wiley & Sons, 2014). 2 D. Liu and C. Svensson, IEEE J. Solid-State Circuits 29, 663 (1994). 3 G. Liu, B.R. Tuttle, and S. Dhar, Appl. Phys. Rev. 2, 021307 (2015). 4 S. Das, T. Isaacs-Smith, A. Ahyi, M.A. Kuroda, and S. Dhar, J. Appl. Phys. 130, 225701 (2021). 5 A. Chanthaphan, T. Hosoi, T. Shimura, and H. Watanabe, AIP Adv. 5, 097134 (2015). 6 K. Tachiki and T. Kimoto, IEEE Trans. Electron Devices 68, 638 (2021). 7 L. Wang, S. Dhar, L.C. Feldman, and M.A. Kuroda, Phys. Status Solidi B 259, 2100224 (2022). Figure 1
更多查看译文
AI 理解论文
溯源树
样例
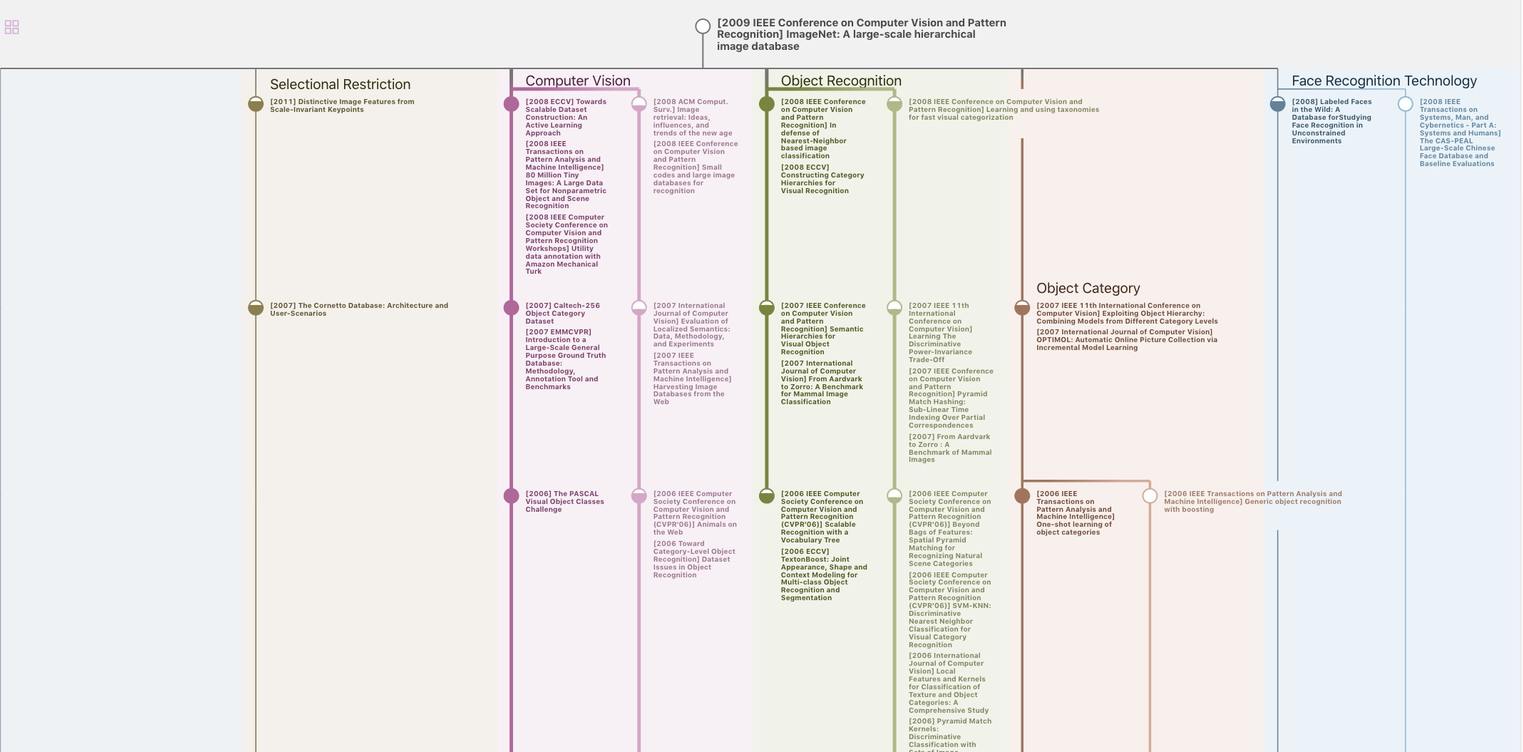
生成溯源树,研究论文发展脉络
Chat Paper
正在生成论文摘要