The major trimeric antenna complexes serve as a site for qH-energy dissipation in plants
Journal of Biological Chemistry(2022)
摘要
Plants and algae are faced with a conundrum: harvesting sufficient light to drive their metabolic needs while dissipating light in excess to prevent photodamage, a process known as nonphotochemical quenching. A slowly relaxing form of energy dissipation, termed qH, is critical for plants’ survival under abiotic stress; however, qH location in the photosynthetic membrane is unresolved. Here, we tested whether we could isolate subcomplexes from plants in which qH was induced that would remain in an energy-dissipative state. Interestingly, we found that chlorophyll (Chl) fluorescence lifetimes were decreased by qH in isolated major trimeric antenna complexes, indicating that they serve as a site for qH-energy dissipation and providing a natively quenched complex with physiological relevance to natural conditions. Next, we monitored the changes in thylakoid pigment, protein, and lipid contents of antenna with active or inactive qH but did not detect any evident differences. Finally, we investigated whether specific subunits of the major antenna complexes were required for qH but found that qH was insensitive to trimer composition. Because we previously observed that qH can occur in the absence of specific xanthophylls, and no evident changes in pigments, proteins, or lipids were detected, we tentatively propose that the energy-dissipative state reported here may stem from Chl–Chl excitonic interaction. Plants and algae are faced with a conundrum: harvesting sufficient light to drive their metabolic needs while dissipating light in excess to prevent photodamage, a process known as nonphotochemical quenching. A slowly relaxing form of energy dissipation, termed qH, is critical for plants’ survival under abiotic stress; however, qH location in the photosynthetic membrane is unresolved. Here, we tested whether we could isolate subcomplexes from plants in which qH was induced that would remain in an energy-dissipative state. Interestingly, we found that chlorophyll (Chl) fluorescence lifetimes were decreased by qH in isolated major trimeric antenna complexes, indicating that they serve as a site for qH-energy dissipation and providing a natively quenched complex with physiological relevance to natural conditions. Next, we monitored the changes in thylakoid pigment, protein, and lipid contents of antenna with active or inactive qH but did not detect any evident differences. Finally, we investigated whether specific subunits of the major antenna complexes were required for qH but found that qH was insensitive to trimer composition. Because we previously observed that qH can occur in the absence of specific xanthophylls, and no evident changes in pigments, proteins, or lipids were detected, we tentatively propose that the energy-dissipative state reported here may stem from Chl–Chl excitonic interaction. Photosynthetic organisms possess pigment–protein antenna complexes, which can switch from a light-harvesting state to an energy-dissipating state (1Liguori N. Periole X. Marrink S.J. Croce R. From light-harvesting to photoprotection: structural basis of the dynamic switch of the major antenna complex of plants (LHCII).Sci. Rep. 2015; 515661Crossref PubMed Scopus (100) Google Scholar, 2Valkunas L. Chmeliov J. Krüger T.P.J. Ilioaia C. van Grondelle R. How photosynthetic proteins switch.J. Phys. Chem. Lett. 2012; 3: 2779-2784Crossref Scopus (51) Google Scholar). This switching capability regulates how much light is directed toward photochemistry and ultimately how much carbon dioxide is fixed by photosynthesis (3Zhu X.G. Long S.P. Ort D.R. Improving photosynthetic efficiency for greater yield.Annu. Rev. Plant Biol. 2010; 61: 235-261Crossref PubMed Scopus (1243) Google Scholar). The fine-tuning of light energy usage is achieved at the molecular level by proteins that act at or around these pigment–protein complexes (4Demmig-Adams B. Garab G. Adams III, W. Govindjee Non-photochemical Quenching and Energy Dissipation in Plants, Algae and Cyanobacteria. Springer, Dordrecht2014Crossref Google Scholar). Understanding the regulatory mechanisms involved in the protection against excess light, or photoprotection, has important implications for engineering optimized light-use efficiency in plants (5Bailey-Serres J. Parker J.E. Ainsworth E.A. Oldroyd G.E.D. Schroeder J.I. Genetic strategies for improving crop yields.Nature. 2019; 575: 109-118Crossref PubMed Scopus (595) Google Scholar) and thereby increasing crop productivity when light reactions are limiting such as upon transition from sun to shade (6Kromdijk J. Glowacka K. Leonelli L. Gabilly S.T. Iwai M. Niyogi K.K. et al.Improving photosynthesis and crop productivity by accelerating recovery from photoprotection.Science. 2016; 354: 857-861Crossref PubMed Scopus (789) Google Scholar, 7Wang Y. Burgess S.J. de Becker E.M. Long S.P. Photosynthesis in the fleeting shadows: an overlooked opportunity for increasing crop productivity?.Plant J. 2020; 101: 874-884Crossref PubMed Scopus (47) Google Scholar, 8Zhu X.G. Ort D.R. Whitmarsh J. Long S.P. The slow reversibility of photosystem II thermal energy dissipation on transfer from high to low light may cause large losses in carbon gain by crop canopies: a theoretical analysis.J. Exp. Bot. 2004; 55: 1167-1175Crossref PubMed Scopus (223) Google Scholar) and/or tolerance to photo-oxidative stress in suboptimal environments (9Murchie E.H. Niyogi K.K. Manipulation of photoprotection to improve plant photosynthesis.Plant Physiol. 2011; 155: 86-92Crossref PubMed Scopus (342) Google Scholar). Nonphotochemical quenching (NPQ) processes protect photosynthetic organisms by safely dissipating excess absorbed light energy as heat and is assessed as a decrease of chlorophyll (Chl) fluorescence (10Horton P. Ruban A.V. Walters R.G. Regulation of light harvesting in green plants.Annu. Rev. Plant Physiol. Plant Mol. Biol. 1996; 47: 655-684Crossref PubMed Scopus (1429) Google Scholar). Indeed absorbed light energy by Chl can fuel photosynthetic reaction (photochemistry), be re-emitted as heat or as fluorescence (11Müller P. Li X.P. Niyogi K.K. Non-photochemical quenching. A response to excess light energy.Plant Physiol. 2001; 125: 1558-1566Crossref PubMed Scopus (2166) Google Scholar). Upon blocking photochemistry using a saturating pulse of light, maximum fluorescence (Fm) is measured and inversely correlated with the amount of energy dissipated by NPQ (12Brooks M.D. Niyogi K.K. Use of a pulse-amplitude modulated chlorophyll fluorometer to study the efficiency of photosynthesis in Arabidopsis plants.Methods Mol. Biol. 2011; 775: 299-310Crossref PubMed Scopus (36) Google Scholar, 13Chukhutsina V.U. Holzwarth A.R. Croce R. Time-resolved fluorescence measurements on leaves: principles and recent developments.Photosynth. Res. 2019; 140: 355-369Crossref PubMed Scopus (25) Google Scholar). Several NPQ mechanisms have been described and classified based on their recovery kinetics and/or molecular players involved (14Bassi R. Dall'Osto L. Dissipation of light energy absorbed in excess: the molecular mechanisms.Annu. Rev. Plant Biol. 2021; 72: 47-76Crossref PubMed Scopus (53) Google Scholar, 15Malnoë A. Photoinhibition or photoprotection of photosynthesis? Update on the (newly termed) sustained quenching component, qH.Environ. Exp. Bot. 2018; 154: 123-133Crossref Scopus (93) Google Scholar, 16Pinnola A. Bassi R. Molecular mechanisms involved in plant photoprotection.Biochem. Soc. Trans. 2018; 46: 467-482Crossref PubMed Scopus (122) Google Scholar): qE, qZ, qH, qI, qT with the letter “q” referring to quenching, that is, decrease of fluorescence, followed by a letter specifying the mechanism. In plants, the rapidly reversible NPQ (relaxes within minutes), or flexible energy dissipation mode, qE, relies on ΔpH, the protein PsbS, and the xanthophyll pigment zeaxanthin (17Niyogi K.K. Truong T.B. Evolution of flexible non-photochemical quenching mechanisms that regulate light harvesting in oxygenic photosynthesis.Curr. Opin. Plant Biol. 2013; 16: 307-314Crossref PubMed Scopus (366) Google Scholar). The slowly reversible NPQ (relaxes within hours to days), or sustained energy dissipation mode, includes several mechanisms such as qZ (zeaxanthin dependent, ΔpH independent (18Dall'Osto L. Caffarri S. Bassi R. A mechanism of nonphotochemical energy dissipation, independent from PsbS, revealed by a conformational change in the antenna protein CP26.Plant Cell. 2005; 17: 1217-1232Crossref PubMed Scopus (206) Google Scholar)), qH (see later and Ref. (15Malnoë A. Photoinhibition or photoprotection of photosynthesis? Update on the (newly termed) sustained quenching component, qH.Environ. Exp. Bot. 2018; 154: 123-133Crossref Scopus (93) Google Scholar) for a review), and qI (due to photosystem II [PSII] reaction center subunit D1 photoinactivation (19Krause G.H. Somersalo S. Zumbusch E. Weyers B. Laasch H. On the mechanism of photoinhibition in chloroplasts. Relationship between changes in fluorescence and activity of photosystem II.J. Plant Physiol. 1990; 136: 472-479Crossref Scopus (85) Google Scholar), which can be reversed by D1 repair (20Nawrocki W.J. Liu X. Raber B. Hu C. de Vitry C. Bennett D.I.G. et al.Molecular origins of induction and loss of photoinhibition-related energy dissipation qI.Sci. Adv. 2021; 7eabj0055Crossref PubMed Scopus (12) Google Scholar)). Energy redistribution through qT is due to state transition, the movement of antenna phosphorylated by the kinase STN7 (21Quick W.P. Stitt M. An examination of factors contributing to non-photochemical quenching of chlorophyll fluorescence in barley leaves.Biochim. Biophys. Acta. 1989; 977: 287-296Crossref Scopus (248) Google Scholar). We have recently uncovered, using chemical mutagenesis and genetic screens in Arabidopsis thaliana, several molecular players regulating a slowly reversible NPQ mechanism, which we named qH (22Amstutz C.L. Fristedt R. Schultink A. Merchant S.S. Niyogi K.K. Malnoë A. An atypical short-chain dehydrogenase-reductase functions in the relaxation of photoprotective qH in Arabidopsis.Nat. Plants. 2020; 6: 154-166Crossref PubMed Scopus (24) Google Scholar, 23Brooks M.D. Sylak-Glassman E.J. Fleming G.R. Niyogi K.K. A thioredoxin-like/beta-propeller protein maintains the efficiency of light harvesting in Arabidopsis.Proc. Natl. Acad. Sci. U. S. A. 2013; 110: 2733-2740Crossref Scopus (70) Google Scholar, 24Bru P. Nanda S. Malnoë A. A genetic screen to identify new molecular players involved in photoprotection qH in Arabidopsis thaliana.Plants. 2020; 9: 1565Crossref Scopus (5) Google Scholar, 25Malnoë A. Schultink A. Shahrasbi S. Rumeau D. Havaux M. Niyogi K.K. The plastid lipocalin LCNP is required for sustained photoprotective energy dissipation in Arabidopsis.Plant Cell. 2018; 30: 196-208Crossref PubMed Scopus (77) Google Scholar). qH requires the plastid lipocalin (LCNP) (25Malnoë A. Schultink A. Shahrasbi S. Rumeau D. Havaux M. Niyogi K.K. The plastid lipocalin LCNP is required for sustained photoprotective energy dissipation in Arabidopsis.Plant Cell. 2018; 30: 196-208Crossref PubMed Scopus (77) Google Scholar) for its induction or for its activation, is negatively regulated by suppressor of quenching 1 (SOQ1) (23Brooks M.D. Sylak-Glassman E.J. Fleming G.R. Niyogi K.K. A thioredoxin-like/beta-propeller protein maintains the efficiency of light harvesting in Arabidopsis.Proc. Natl. Acad. Sci. U. S. A. 2013; 110: 2733-2740Crossref Scopus (70) Google Scholar, 26Yu G. Hao J. Pan X. Shi L. Zhang Y. Wang J. et al.Structure of Arabidopsis SOQ1 lumenal region unveils C-terminal domain essential for negative regulation of photoprotective qH.Nat. Plants. 2022; 8: 840-855Crossref PubMed Scopus (4) Google Scholar), and is inactivated by relaxation of qH 1 (ROQH1) (22Amstutz C.L. Fristedt R. Schultink A. Merchant S.S. Niyogi K.K. Malnoë A. An atypical short-chain dehydrogenase-reductase functions in the relaxation of photoprotective qH in Arabidopsis.Nat. Plants. 2020; 6: 154-166Crossref PubMed Scopus (24) Google Scholar). Importantly, qH is independent of PsbS, ΔpH, xanthophyll pigments, and phosphorylation by STN7 (23Brooks M.D. Sylak-Glassman E.J. Fleming G.R. Niyogi K.K. A thioredoxin-like/beta-propeller protein maintains the efficiency of light harvesting in Arabidopsis.Proc. Natl. Acad. Sci. U. S. A. 2013; 110: 2733-2740Crossref Scopus (70) Google Scholar, 25Malnoë A. Schultink A. Shahrasbi S. Rumeau D. Havaux M. Niyogi K.K. The plastid lipocalin LCNP is required for sustained photoprotective energy dissipation in Arabidopsis.Plant Cell. 2018; 30: 196-208Crossref PubMed Scopus (77) Google Scholar). Strikingly, when qH is constitutively active in a soq1 roqh1 mutant, plants are severely light limited and display a stunted phenotype (22Amstutz C.L. Fristedt R. Schultink A. Merchant S.S. Niyogi K.K. Malnoë A. An atypical short-chain dehydrogenase-reductase functions in the relaxation of photoprotective qH in Arabidopsis.Nat. Plants. 2020; 6: 154-166Crossref PubMed Scopus (24) Google Scholar). If qH cannot occur (as in an lcnp mutant), a higher amount of lipid peroxidation is observed, and plants are severely light damaged under stress conditions such as cold temperature and high light (HL) (25Malnoë A. Schultink A. Shahrasbi S. Rumeau D. Havaux M. Niyogi K.K. The plastid lipocalin LCNP is required for sustained photoprotective energy dissipation in Arabidopsis.Plant Cell. 2018; 30: 196-208Crossref PubMed Scopus (77) Google Scholar, 27Levesque-Tremblay G. Havaux M. Ouellet F. The chloroplastic lipocalin AtCHL prevents lipid peroxidation and protects Arabidopsis against oxidative stress.Plant J. 2009; 60: 691-702Crossref PubMed Scopus (71) Google Scholar). Our present working hypothesis is that, under stress conditions, LCNP binds or modifies a molecule in the vicinity of or within the antenna proteins, thereby triggering a conformational change that converts antenna proteins from a light-harvesting to a dissipative state. In WT Arabidopsis plants, qH occurs in response to cold and HL (25Malnoë A. Schultink A. Shahrasbi S. Rumeau D. Havaux M. Niyogi K.K. The plastid lipocalin LCNP is required for sustained photoprotective energy dissipation in Arabidopsis.Plant Cell. 2018; 30: 196-208Crossref PubMed Scopus (77) Google Scholar), whereas the soq1 mutant can display qH under nonstress conditions upon a 10 min HL treatment (23Brooks M.D. Sylak-Glassman E.J. Fleming G.R. Niyogi K.K. A thioredoxin-like/beta-propeller protein maintains the efficiency of light harvesting in Arabidopsis.Proc. Natl. Acad. Sci. U. S. A. 2013; 110: 2733-2740Crossref Scopus (70) Google Scholar). In plants, the peripheral antenna of PSII is composed of pigment-binding, light-harvesting complex (Lhcb) proteins, which are divided into minor subunits (Lhcb4, Lhcb5, Lhcb6, or CP29, CP26, CP24, respectively) present as monomers and major subunits (Lhcb1, Lhcb2, and Lhcb3) also referred to as LHCII, forming heterotrimeric and homotrimeric complexes associated to PSII in a strongly, moderately, or loosely bound manner (28Ballottari M. Girardon J. Dall'osto L. Bassi R. Evolution and functional properties of photosystem II light harvesting complexes in eukaryotes.Biochim. Biophys. Acta. 2012; 1817: 143-157Crossref PubMed Scopus (128) Google Scholar, 29Crepin A. Caffarri S. Functions and evolution of Lhcb isoforms composing LHCII, the major light harvesting complex of photosystem II of green eukaryotic organisms.Curr. Protein Pept. Sci. 2018; 19: 699-713Crossref PubMed Scopus (36) Google Scholar). The pigments associated with the major and minor antenna complexes include Chls a and b and xanthophylls, such as lutein, violaxanthin, zeaxanthin, and neoxanthin (30Jahns P. Holzwarth A.R. The role of the xanthophyll cycle and of lutein in photoprotection of photosystem II.Biochim. Biophys. Acta. 2012; 1817: 182-193Crossref PubMed Scopus (795) Google Scholar). The mutant chlorina1 does not accumulate trimeric Lhcbs because it lacks Chl b, but it does accumulate some monomeric Lhcbs with Chl a only (31Takabayashi A. Kurihara K. Kuwano M. Kasahara Y. Tanaka R. Tanaka A. The oligomeric states of the photosystems and the light-harvesting complexes in the Chl b-less mutant.Plant Cell Physiol. 2011; 52: 2103-2114Crossref PubMed Scopus (35) Google Scholar). qH is no longer observed in the double mutant soq1 chlorina 1 (25Malnoë A. Schultink A. Shahrasbi S. Rumeau D. Havaux M. Niyogi K.K. The plastid lipocalin LCNP is required for sustained photoprotective energy dissipation in Arabidopsis.Plant Cell. 2018; 30: 196-208Crossref PubMed Scopus (77) Google Scholar), indicating that qH may require the trimeric antenna and/or Chl b. Here, we investigated whether qH remained active upon isolation of thylakoids or photosynthetic subcomplexes with aim to narrow down the location of the qH quenching site and characterize its properties. We measured the Chl fluorescence lifetimes of intact leaves, isolated thylakoids, and isolated complexes from plants (WT and several mutants relating to qH) exposed to nonstress or stress conditions with active or inactive qH. Isolation of partly quenched LHCII directly from thylakoid membranes with active qH showed that qH can occur in the major trimeric LHCII complexes. Through genome editing and genetic crosses, we further demonstrated that qH does not rely on a specific major Lhcb subunit, suggesting that qH is not because of specific amino acid variation among Lhcb1, Lhcb2, and Lhcb3 (such as phosphorylation in Lhcb1 and Lhcb2 or the presence of cysteine in Lhcb2.3 or aromatic residues in Lhcb3) and/or that compensation from other major Lhcb proteins may occur. Prior to this work, only a few studies had reported a quenched conformation of isolated LHCII trimers, and in contrast to the native isolation reported here, quenching was achieved in vitro, after full solubilization of LHCII (32Ilioaia C. Johnson M.P. Horton P. Ruban A.V. Induction of efficient energy dissipation in the isolated light-harvesting complex of photosystem II in the absence of protein aggregation.J. Biol. Chem. 2008; 283: 29505-29512Abstract Full Text Full Text PDF PubMed Scopus (91) Google Scholar, 33van Oort B. van Hoek A. Ruban A.V. van Amerongen H. Equilibrium between quenched and nonquenched conformations of the major plant light-harvesting complex studied with high-pressure time-resolved fluorescence.J. Phys. Chem. B. 2007; 111: 7631-7637Crossref PubMed Scopus (62) Google Scholar). Successful isolation of natively quenched LHCII paves the way for revealing its molecular origin. Previously, we demonstrated that qH is induced by a cold and HL treatment on whole plants of Arabidopsis in mutants and importantly also in WT. We found that the amount of NPQ measured by Chl fluorescence imaging can reach a high level, approximately 12 in the soq1 mutant, and this induction of NPQ is LCNP dependent as it does not occur in the soq1 lcnp double mutant (25Malnoë A. Schultink A. Shahrasbi S. Rumeau D. Havaux M. Niyogi K.K. The plastid lipocalin LCNP is required for sustained photoprotective energy dissipation in Arabidopsis.Plant Cell. 2018; 30: 196-208Crossref PubMed Scopus (77) Google Scholar). We also observed constitutive qH from nontreated plants in the soq1 roqh1 double mutant, which displayed Fm values ∼85% lower than WT or soq1 roqh1 lcnp, indicating a high NPQ yield (22Amstutz C.L. Fristedt R. Schultink A. Merchant S.S. Niyogi K.K. Malnoë A. An atypical short-chain dehydrogenase-reductase functions in the relaxation of photoprotective qH in Arabidopsis.Nat. Plants. 2020; 6: 154-166Crossref PubMed Scopus (24) Google Scholar). To ascertain that qH under stress condition such as cold and HL, or in the double mutant soq1 roqh1, was due to a decrease in Chl excited-state lifetime, we measured fluorescence lifetime via time-correlated single photon counting on both intact leaves and thylakoids isolated from plants cold and HL treated or nontreated. Here, we used the laser at a saturating light intensity to close PSII reaction centers so that differences in lifetime can be attributed to NPQ (34Sylak-Glassman E.J. Zaks J. Amarnath K. Leuenberger M. Fleming G.R. Characterizing non-photochemical quenching in leaves through fluorescence lifetime snapshots.Photosynth. Res. 2016; 127: 69-76Crossref PubMed Scopus (19) Google Scholar). Strikingly, nontreated soq1 roqh1 indeed displayed a decreased amplitude-weighted average fluorescence lifetimes (τavg) compared with controls (Fig. 1, light gray bars), with a much shorter value in both leaves (∼0.1 ns versus ∼1.5 ns) and thylakoids (∼0.2 ns versus ∼1.1 ns). These data unequivocally show that LCNP-dependent NPQ, qH, promotes a Chl de-excitation pathway, which remains active upon isolation of thylakoid membranes. Next, we exposed plants to a 6 h cold and HL treatment (6 °C and 1500 μmol photons m−2 s−1) followed by dark acclimation for 5 min to relax qE. During this treatment, qH is induced and so is qZ as zeaxanthin accumulates (de-epoxidation state value of approximately 0.7 [stress] versus 0.05 [nonstress] in all lines (22Amstutz C.L. Fristedt R. Schultink A. Merchant S.S. Niyogi K.K. Malnoë A. An atypical short-chain dehydrogenase-reductase functions in the relaxation of photoprotective qH in Arabidopsis.Nat. Plants. 2020; 6: 154-166Crossref PubMed Scopus (24) Google Scholar, 25Malnoë A. Schultink A. Shahrasbi S. Rumeau D. Havaux M. Niyogi K.K. The plastid lipocalin LCNP is required for sustained photoprotective energy dissipation in Arabidopsis.Plant Cell. 2018; 30: 196-208Crossref PubMed Scopus (77) Google Scholar)); the remaining slowly relaxing quenching processes are grouped under the term qI and are in part due to photoinactivation of PSII. In Figure 1, colored bars show that the cold and HL treatment on plants results in a decreased Chl excited-state lifetime in both leaves and isolated thylakoids. Interestingly, cold and HL-treated soq1 leaves displayed τavg values similar to nontreated soq1 roqh1 indicating that this stress treatment triggers a fully active qH state in soq1. WT leaves displayed an intermediate τavg value between active qH (soq1) and inactive qH (soq1 roqh1 lcnp), further establishing the occurrence, and physiological relevance, of qH in promoting energy dissipation under abiotic stress (Fig. 1A). The calculated NPQτ derived from the τavg values were all in agreement with the NPQ values measured by pulse amplitude–modulated Chl fluorescence (22Amstutz C.L. Fristedt R. Schultink A. Merchant S.S. Niyogi K.K. Malnoë A. An atypical short-chain dehydrogenase-reductase functions in the relaxation of photoprotective qH in Arabidopsis.Nat. Plants. 2020; 6: 154-166Crossref PubMed Scopus (24) Google Scholar, 25Malnoë A. Schultink A. Shahrasbi S. Rumeau D. Havaux M. Niyogi K.K. The plastid lipocalin LCNP is required for sustained photoprotective energy dissipation in Arabidopsis.Plant Cell. 2018; 30: 196-208Crossref PubMed Scopus (77) Google Scholar) with 11, 3.3, and 2 for soq1, WT, and soq1 roqh1 lcnp, respectively; soq1 lcnp was not measured here as its phenotype is similar to soq1 roqh1 lcnp. We also measured the fluorescence lifetime from leaves of soq1 npq4 roqh1 (lacks SOQ1, PsbS, and ROQH1), soq1 roqh1 ROQH1 overexpressor (OE), and several mutant alleles of roqh1 and soq1 roqh1 (Fig. S1 and Table S1). The τavg values, and calculated NPQτ, further highlighted that qH is independent of PsbS (soq1 npq4 roqh1 has a low τavg in a similar range to soq1 roqh1, ∼60 ps versus 130 ps) and that ROQH1 is required for relaxation of qH (NPQτ of roqh1 mutant and soq1 roqh1 ROQH1 OE, respectively, higher and lower than WT). Of note, in thylakoids isolated from cold and HL-treated plants, the τavg values were overall higher than observed in intact leaves, and the difference in τavg between WT and soq1 roqh1 lcnp was no longer apparent (Fig. 1B), whereas the τavg values of isolated thylakoids from nontreated plants without qH (soq1, WT, and soq1 roqh1 lcnp) were all lower than observed in intact leaves. Therefore, possible changes in, for example, membrane macro-organization, protein content, or complexes occurring during thylakoid isolation have an opposite effect on fluorescence lifetime depending on the starting state (active or inactive NPQ) for reasons we cannot explain. We probed the release of Chl fluorescence by step solubilization of thylakoid membrane preparation (Fig. S2), and it showed that qH is partly due to protein–protein and lipid–protein interactions in the membrane (cold and HL, Qm soq1 higher than soq1 roqh1 lcnp) and due to pigment–protein interactions (Qpi also higher), which may explain the lower NPQτ of soq1 thylakoids compared with leaves (and longer τavg of soq1 roqh1 thylakoids compared with leaves) as some of these interactions may have been lost during thylakoid preparation. Yet, although smaller, the retention of active qH in soq1 thylakoids offered a unique opportunity to explore whether quenched photosynthetic subcomplexes could be isolated. Next, we tested whether we could observe qH in a specific isolated pigment–protein complex. The lines soq1 (active qH) and soq1 lcnp (inactive qH) were chosen for this purpose (soq1 roqh1 and soq1 roqh1 lcnp could have been used, but soq1 roqh1 plants are much smaller because of light limitation by constitutive active qH). Plants were treated with cold and HL for 6 h at 6 °C and 1500 μmol photons m−2 s−1 followed by dark acclimation for 5 min to relax qE. Thylakoids were isolated, solubilized, and fractionated by gel filtration to separate complexes based on their size. The separation profiles of photosynthetic complexes were similar for soq1 and soq1 lcnp (Fig. S3A). Fractions corresponding to PSII–LHCII megacomplexes, supercomplexes, PSI–LHCI supercomplexes, PSII core dimer, LHCII trimer, and LHCII/Lhcb monomer (containing both major and minor antenna, see later) as well as smaller fractions (peaks 7 and 8) were collected, and their relative fluorescence yield was measured by video imaging (Fig. S3B). The LHCII trimer fraction clearly displayed a lower relative fluorescence yield with active qH. Room-temperature fluorescence spectra were measured at the same low Chl concentration (0.1 μg ml−1) to prevent reabsorption and with excitation at 625 nm (isosbestic point) to excite both Chls a and b equally; the Chl a/b ratio is similar between the compared samples so absorption at 625 nm should be equal. Complexes from nontreated WT were isolated for reference; material came from plants grown under standard light conditions. The LHCII trimer fraction displayed a relative fluorescence yield at 680 nm that was on average 24% ± 8% lower with active qH compared with inactive qH and WT reference, whereas the LHCII/Lhcb monomer fraction displayed no significant differences among samples (Figs. 2A and S4, A and B). A complementary approach separating pigment–protein complexes following solubilization by clear native-PAGE further evidenced that qH is active in isolated LHCII trimers (Figs. 2B and S4C). These results suggest that qH occurs at least partly in the LHCII trimer and remains active even after isolation of the solubilized protein complex. We measured the Chl fluorescence lifetimes of LHCII trimer, LHCII/Lhcb monomer, and PSII dimer fractions. We observed in the active qH LHCII trimer fraction a ∼20% shorter τavg compared with that of inactive qH (∼2.6 ns for soq1 versus ∼3.3 ns for soq1 lcnp) in agreement with the ∼20% decrease in relative fluorescence yield (Fig. 3); for reference, nontreated WT LHCII τavg is ∼3.1 ns (Table S2). No differences in fluorescence lifetimes caused by qH were detected in either the LHCII/Lhcb monomer or PSII dimer fractions. These results unambiguously demonstrate that qH promotes a Chl de-excitation pathway in the trimeric antenna and is distinct from qI. In our previous work, the question persisted whether qH was antenna dependent as we had not shown direct evidence of quenching in the antenna. We examined the pigment, lipid, and protein content by HPLC, LC–MS, and SDS-PAGE, respectively, to investigate which molecular changes may be at the origin of the qH-energy dissipative state in the trimeric antenna. There were no apparent differences in pigment composition (Fig. S5A) or abundance (Fig. S5B) in LHCII trimers from active or inactive qH. Composition of the main chloroplastic lipids in LHCII trimer, LHCII/Lhcb monomer, or thylakoid extracts indicated no significant differences (Figs. S6 and S7); the distribution of thylakoid lipids is in line with the literature (35Burgos A. Szymanski J. Seiwert B. Degenkolbe T. Hannah M.A. Giavalisco P. et al.Analysis of short-term changes in the Arabidopsis thaliana glycerolipidome in response to temperature and light.Plant J. 2011; 66: 656-668Crossref PubMed Scopus (107) Google Scholar). Of note, the 6 h cold and HL treatment did not alter the lipid profile significantly. The protein content was also similar in LHCII trimer from active or inactive qH (with an equivalent low amount of minor monomeric Lhcb4), and there were no visible additional protein bands or size shifts (Fig. S8). Investigation of possible post-translational modifications of amino acid residues by protein mass spectrometry will be the subject of future work (preliminary exploration did not show evident changes). We observed LHCII subunits in the monomer fractions (probed with anti-Lhcb2), hence the “LHCII/Lhcb” name, with a higher content in the cold and HL-treated samples compared with nontreated WT; this could be due to monomerization of trimers during the cold and HL treatment. Having gained the knowledge that qH partly occurs in the LHCII trimer, the next question was whether a specific LHCII subunit would be required, and this may provide a hint to the molecular origin of qH. We used genetic crosses together with genome editing to combine the soq1 mutation with mutations in LHCII genes. The soq1 mutant was cros
更多查看译文
关键词
photosynthesis,energy dissipation,nonphotochemical quenching qH,Arabidopsis thaliana,light-harvesting complexes,time-resolved fluorescence,CRISPR–Cas9,abiotic stress
AI 理解论文
溯源树
样例
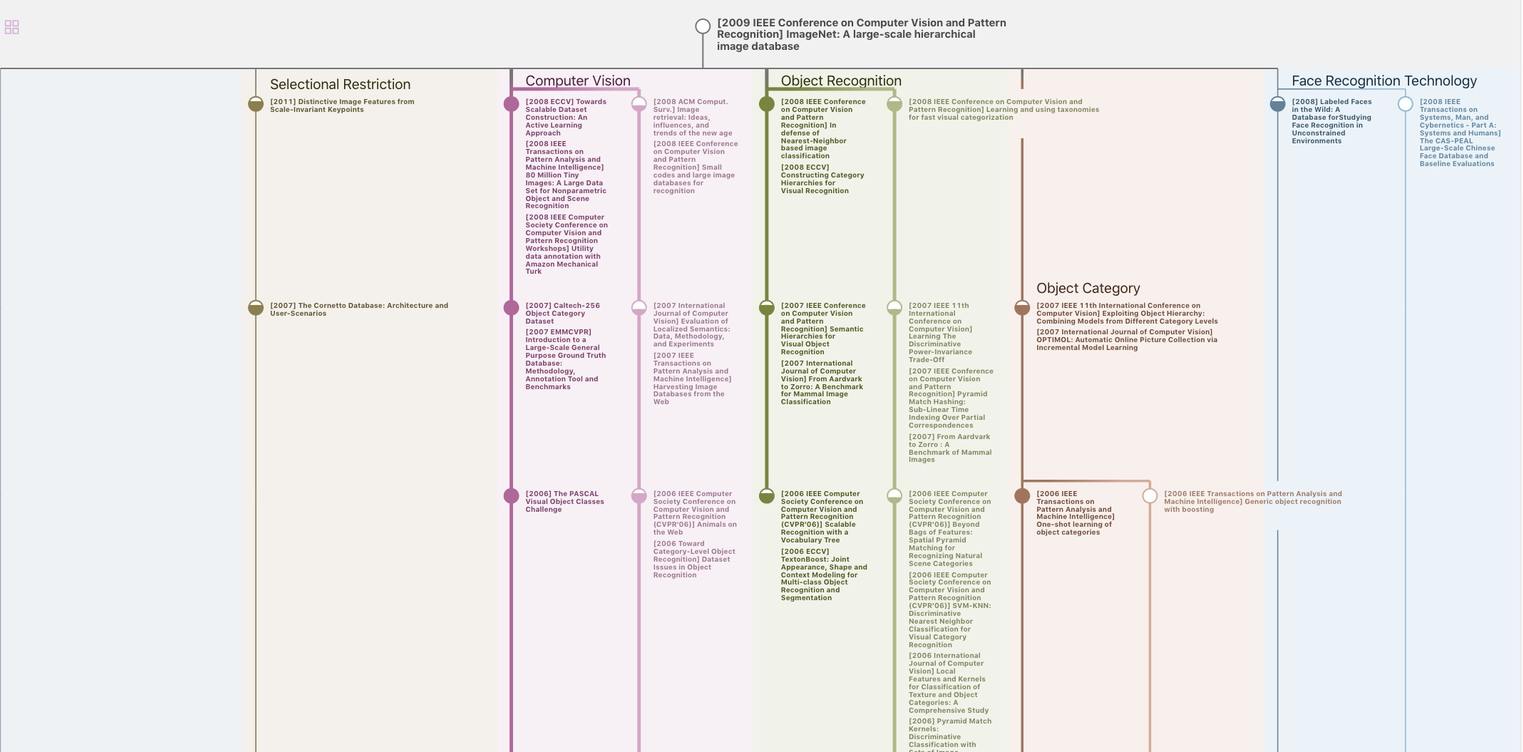
生成溯源树,研究论文发展脉络
Chat Paper
正在生成论文摘要