Oxygen vacancy-induced anomalous Hall effect in a non-magnetic oxide
semanticscholar(2021)
摘要
The anomalous Hall effect, a hallmark of broken time-reversal symmetry and spin-orbit coupling, is frequently observed in magnetically polarized systems. Its realization in non-magnetic systems, however, remains elusive. Here, we report on the observation of anomalous Hall effect in nominally non-magnetic KTaO3. Anomalous Hall effect emerges in reduced KTaO3 and shows an extrinsic to intrinsic crossover. A paramagnetic behavior is observed in reduced samples using first principles calculations and quantitative magnetometry. The observed anomalous Hall effect follows the oxygen vacancy-induced magnetization response, suggesting that the localized magnetic moments of the oxygen vacancies scatter conduction electrons asymmetrically and give rise to anomalous Hall effect. The anomalous Hall conductivity becomes insensitive to scattering rate in the low temperature limit (T<5 K), implying that the Berry curvature of the electrons on the Fermi surface controls the anomalous Hall effect. Our observations describe a detailed picture of many-body interactions, triggering anomalous Hall effect in a non-magnetic system. Description of the anomalous Hall effect (AHE) of magnetically polarized systems [1] in terms of the Berry curvature of the quasiparticles on the Fermi surface is one of the major accomplishments of modern condensed matter theory [2–7]. The mobile charge carriers gain a transverse momentum in systems with a magnetic polarization and spin–orbit coupling. The built-up Hall voltage, i.e. anomalous Hall component, can be observed as a distinct, additional contribution in the Hall measurements, superposed on the ordinary Hall effect [8–13]. The AHE originates from spin-orbit coupling and is proportional to the magnetization, R B λM B , where λ relates magnetization to the anomalous Hall component [14]. Theoretical [15–17] and experimental [18– 20] observations of defect-induced magnetization were previously reported in non-magnetic oxides. Experimental realization of anomalous Hall effect, however, remains elusive in these systems. The degree of disorder within a system determines the nature of the AHE response. The intrinsic AHE occurs in the moderately dirty regime in which anomalous Hall conductivity becomes nondissipative, i.e. independent of the scattering rate [21]. In this regime, the Berry phase of the quasiparticles on the Fermi surface acts as an effective magnetic field generating a transverse momentum. In the dirty regime, however, the extrinsic anomalous Hall conductivity is directly proportional to the longitudinal conductivity, σ ∝ σ . , and asymmetric scattering of the conduction electrons gives rise to AHE. The extrinsic to intrinsic crossover occurs when the scattering rate becomes comparable to Fermi energy [22]. In clean regime, an extrinsic anomalous Hall response emerges due to skew scattering of charge carriers and shows a linear relationship with longitudinal conductivity, σ ∝ σ . KTaO3 is an incipient ferroelectric [23] in which a combination of a small effective mass of electrons and large dielectric constant, i.e. giant effective Bohr radius [24], induces a metallic state even in the dilute doping regimes. The KTaO3 conduction states are derived from Ta 5d and have higher mobility and spin-orbit coupling compared to Ti 3d states in SrTiO3 [25,26]. Spin-orbit coupling lifts the degeneracy of Ta 5d states and splits them into J=3/2 and J=1/2 with a 0.4 eV energy gap, where J is total angular momentum [27]. Here, we report on the observation of an anomalous Hall signal in reduced KTaO3. An extrinsic AHE emerges at low temperature (T<60 K). Our observations suggest that oxygen vacancies play the role of paramagnetic point defects, scattering the conduction electrons and giving rise to AHE. The anomalous Hall conductivity becomes independent of the scattering rate below 5 K, suggesting an extrinsic to intrinsic AHE crossover. Oxygen vacancies were introduced to KTaO3 (100) single crystal substrates by thermal annealing in ultra-high vacuum using a molecular beam epitaxy system. A 10 nm TiOx was deposited at 800 °C and 1×10-9 Torr on the surface of KTaO3. Here, the TiOx layer acts as an oxygen getter and capping layer during annealing. The substrate became dark due to introduction of oxygen vacancies. The temperature dependent magneto-transport measurements were performed using a Quantum Design physical property measurement system (PPMS) with a lock-in amplifier (SR830, Stanford Research Systems) in AC mode with an excitation current of 10 μA and a frequency of 13.33 Hz. Magneto-transport measurements were performed using a Van der Pauw configuration. Gold contacts were deposited using a sputter system at the corners of the samples through a shadow mask. The Hall experiments were carried out at different temperatures (Supplementary materials, S1). The temperature-dependent Hall carrier densities extracted, n 1 eR ⁄ , where R is the Hall coefficient and e is the elementary charge. The Hall coefficient, R dR dB ⁄ , was extracted from a linear fit to the transverse resistance at a magnetic field range where AHE is saturated. Magnetization measurements were performed using a Quantum Design Superconducting Quantum Interference Device (SQUID) magnetometer. Magnetization samples were handled specifically to avoid any magnetic contamination. Magneto-transport measurements below 1 K were carried out in a Triton, Oxford Instruments, bottom loading dilution refrigerator. Oxygen vacancies introduce itinerant electrons to Ta 5d derived states. Figure 1(a) shows resistance with temperature (300-0.03 K). The sheet carrier density is 2.5×1015 cm-2 at 60 K and reveals carrier freeze out below 5 K, reaching 4×1014 cm-2 at 30 mK, Fig. 1(b). The residual resistivity ratio (ρ ρ . ⁄ ) is 342, and the carrier mobility increases from ~20 cm2/Vs at room temperature to ~9,000 cm2/Vs at 30 mK due to screening of the longitudinal optical phonons. All the conduction electrons at the anomalous Hall experiment temperatures are from J=3/2, Ta 5d states due to the large spin-orbit coupling gap (0.4 eV [25,27]). KTaO3 shows a relatively large and positive longitudinal magnetoresistance at 30 mK. This contrasts with the Kondo picture of dilute magnetism in which a negative longitudinal magnetoresistance is expected [28,29]. Longitudinal magneto-conductance shows signatures of weak anti-localization (Supplementary materials, S2). 2D electron systems at the surface of KTaO3 demonstrate large coherence length and signatures of weak anti-localization correction [30–32]. Figure 1. Temperatureand magnetic field-dependent electronic transport. (a) Resistance and (b) Hall carrier density in reduced KTaO3 with temperature. The Hall carrier density shows a strong carrier freezeout below 5 K. (c) Longitudinal magnetoresistance at 30 mK. Figure 2 shows the AHE at different temperatures. The raw Hall results were anti-symmetrized to eliminate the longitudinal magnetoresistance contribution, R R B R B 2 ⁄ (Supplementary materials, S1). The ordinary Hall effect was subtracted from the anti-symmetrized Hall to resolve the AHE contribution, R R R B. AHE shows a monotonic increase and eventual saturation with the applied magnetic field. The details of AHE data extraction is described elsewhere [33–35]. A two-carrier fit to Hall measurement cannot explain the AHE response (Supplementary materials, S3). The AHE saturation resistance R . does not change systematically with the temperature at higher temperatures (5-60 K) and shows an abrupt enhancement below 5 K, peaking at 30 mK. The non-vanishing AHE suggests that the mechanism(s) leading to the AHE sustain down to low temperatures. The abrupt enhancement of AHE coincides with a carrier freeze out and suggests a potential change in the AHE mechanism. Figure 2. Anomalous Hall effect in KTaO3. (a) The extracted AHE after subtraction of the ordinary hall component in reduced KTaO3 at different temperatures. The AHE resistance and saturation magnetic field increase below 5 K. KTaO3 is nominally non-magnetic, but AHE could arise when the localized paramagnetic defects, polarized by an applied magnetic field, interact with conduction electrons. The localized magnetic moments polarize conduction electrons’ angular orbital momentum, leading to a nonzero AHE [36]. The AHE vanishes in the absence of an applied magnetic field. The magnetic moments are typically point defects with localized unpaired electrons. Here, oxygen vacancies could play the role of paramagnetic centers. Density Functional Theory (DFT) calculations with hybrid exchange-correlation functionals were performed to determine the magnetic property of the oxygen vacancies. Details of the DFT calculation method are described in the supplementary materials and were used previously to illustrate the point defects in other perovskite oxide systems [37,38]. These calculations identified that the VO1+ defect is stable when the Fermi level is near or above the conduction band minimum, as is the case here, and in this charge state the defect has a magnetic moment of ~1 μ (Supplementary materials, S4). Figure 3. Comparison of the AHE and magnetization in reduced KTaO3. The normalized AHE and magnetization responses in reduced KTaO3 at 3K. The AHE and magnetization should have similar trends with the magnetic field, R B λM B . The magnetization experiments at different temperatures show a paramagnetic signal on top of the diamagnetic response in the reduced KTaO3. The diamagnetic response was isolated from the high field results (5 T更多
查看译文
AI 理解论文
溯源树
样例
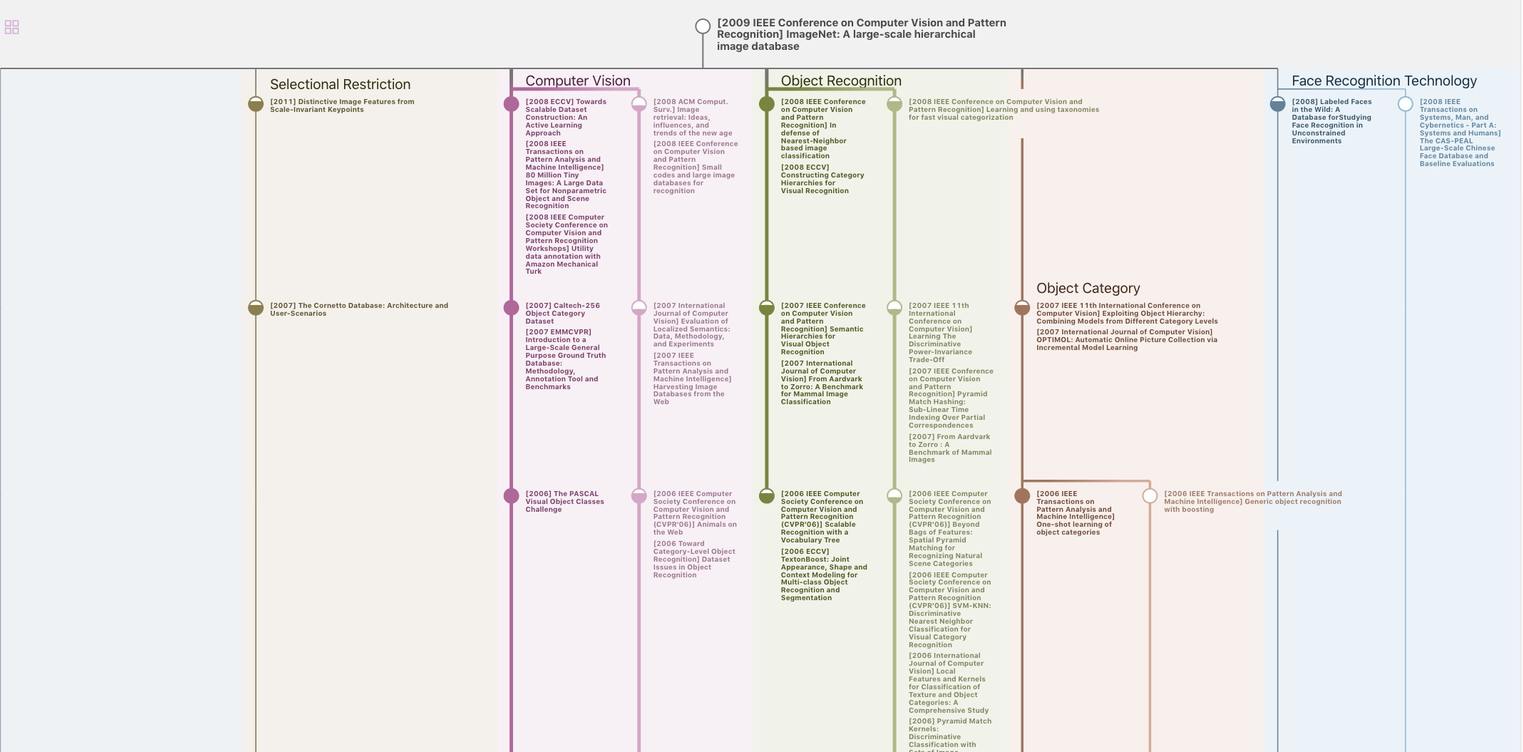
生成溯源树,研究论文发展脉络
Chat Paper
正在生成论文摘要