1-11-2018 Physics of Hollow Bose-Einstein Condensates
semanticscholar(2019)
摘要
Bose-Einstein condensate shells, while occurring in ultracold systems of coexisting phases and potentially within neutron stars, have yet to be realized in isolation on Earth due to the experimental challenge of overcoming gravitational sag. Motivated by the expected realization of hollow condensates by the space-based Cold Atomic Laboratory in microgravity conditions, we study a spherical condensate undergoing a topological change from a filled sphere to a hollow shell. We argue that the collective modes of the system show marked and robust signatures of this hollowing transition accompanied by the appearance of a new boundary. In particular, we demonstrate that the frequency spectrum of the breathing modes shows a pronounced depression as it evolves from the filled sphere limit to the hollowing transition. Furthermore, when the center of the system becomes hollow surface modes show a global restructuring of their spectrum due to the availability of a new, inner, surface for supporting density distortions. We pinpoint universal features of this topological transition as well as analyse the spectral evolution of collective modes in the experimentally relevant case of a bubble-trap. Quantum matter, when subject to transitions of a topological nature, undergoes fundamental changes in its properties [1]. Such transitions involve singular deformations of the underlying space inhabited by the system, be it real or abstract. For instance, the ripping action required to convert a sphere into a torus. In topological materials, which have recently gained prominent attention, matter can transform from being a trivial insulator to one having gapless surface states. The topological nature of the transition can be pinpointed through the calculation or measurement of an abstract Berry phase type global invariant associated with non-trivial winding, for instance, in momentum space [2]. Cold atomic systems, given their spectacular trapping and tuning capabilities, not only enable measuring such topological invariants [3–5], they offer a much more direct, real space version of a topological transition through purely changing physical geometry. As a pioneering instance, the realization of toroidal BoseEinstein condensates (BECs) [6,7] corresponds to a topo(a)kuei.sun@utdallas.edu (b)clannert@smith.edu (c)smivish@illinois.edu logical structure characterized by a homotopy group that is not equivalent to that of a disk. Here we explore salient features associated with the hollowing out of a spherically filled BEC and subsequent formation of a closed, hollow shell. The filled and hollow BECs are topologically inequivalent in that they correspond to different second homotopy groups. In other words, unlike for the filled spherically symmetric BEC, a spherical surface within the hollow BEC that surrounds its center cannot be continuously deformed into a point. In this sense, the hollowing of a condensate corresponds to a topological transition. The study of hollow condensates is particularly germane now in light of the scheduled launch of the Cold Atomic Laboratory (CAL) [8] later this year aimed to investigate ultracold quantum gases aboard the International Space Station. One planned experiment on board involves the first creation of a hollow shell BEC [9,10] using an rf-dressed “bubble trap” potential [11]. While BECs have been produced in a host of interesting geometries [6,7,12–20], gravitational sag has prevented the realization of BEC shells on Earth [21, 22]. Thus, microgravity environments, as expected to be produced in CAL and successp-1 ar X iv :1 61 2. 05 80 9v 3 [ co nd -m at .q ua nt -g as ] 1 4 Ja n 20 18 Karmela Padavić, Kuei Sun, Courtney Lannert, and Smitha Vishveshwara fully demonstrated in the context of BECs in the ZARM drop tower in Germany [23] and in that of normal fluid shells during space shuttle launches [24], are necessary for the realization of hollow condensates. Shell-shaped BECs would be a test bed for quantum fluids in this topology, which naturally occurs in a number of diverse situations from laboratory-based micron scales to astronomical scales. In cold atomic systems, condensate shells are expected in Bose-Fermi mixtures [25–27] and optical-lattice “wedding-cake” structures [28–30] where Mott-insulating regions effectively trap superfluid layers [31]. In neutron stars, extremely high mass densities render ambient relative temperatures low enough for realizing macroscopic quantum states of matter, possibly giving rise to shells of differing states, some corresponding to superconductors and BECs of subatomic particles [32,33]. Collective excitations offer an excellent probe of shape, boundary constraints, and topology, be they in solids, classical liquids, or quantum fluids. For instance, one “hears” the shape of a drum through its normal-mode oscillations [34, 35]. In trapped condensates, the low-lying excitations—their collective modes—were among the first phenomena to be studied after the initial realization of BECs [36–47]. Relevant to our studies, the topological change embodied in the emergence of an additional surface is significant for various physical systems. For example, for ships entering shallow water from the deep seas, the structure of water waves changes when the ocean floor emerges as a relevant boundary [48]. In the aforementioned space shuttle experiments [24] on normal fluid shells, sloshing modes show marked signatures of inner and outer boundaries, akin to those predicted in preliminary theoretical work on BEC shells [49]. Studies of two-dimensional annular BECs [50, 51] have revealed mode spectra that can distinguish an inner boundary in a non-destructive fashion. Such a collective mode analysis becomes even more pertinent for three-dimensional hollow condensates where direct imaging may not discern the presences of a hollow region. Here, we show that the collective mode spectrum presents a natural and powerful way to observe the topological change from filled to hollow in BECs, pinpointing universal features as well as making concrete predictions in experimentally relevant settings. As we discuss in this Letter, the evolution of the collective mode frequencies as a function of a tuning parameter signals the appearance of a new boundary when a condensate undergoes the topological change of hollowing. The effect of the new inner boundary becomes manifest both in collective modes whose oscillations are primarily along the surface of the condensate (surface modes) as well as primarily transverse to it (breathing modes). In the former case of surface modes [Fig. 1(a)], such as high angular momentum modes in a sphere [52], density distortions exponentially decay into the bulk. When the new boundary appears, for any given surface mode, radial distortions are redistributed between the two boundaries. The frequency spectrum shows a sharp jump correspondFig. 1: (Color online) (a) Density variations of surface collective modes in the filled sphere (left), and hollow shell (middle and right), showing the bifurcation of modes confined to the inner and outer surfaces after the system hollows. (b) Density variation of breathing modes through the transition from filled sphere (left) to thin shell (right), showing the localization of mode amplitude to the (hollowing) center of the system during the transition. Surface (radial) modes profiles are presented in a quadrant (full) section of sphere and normalized as in the bar graph. The red solid curves indicate the (Thomas-Fermi) boundaries of the system. ing to this redistribution—each boundary surface hosts fewer nodes of oscillation so the energy associated with a given oscillatory distortion is lowered. For the latter case of transverse modes [Fig. 1(b)], of which the simplest is the spherically symmetric breathing mode [49, 53], the collective mode spectrum shows a distinct dip in frequency when the inner surface is created. Breathing modes localize near the new boundary as the condensate begins to hollow. Density and stiffness of the condensate in the center of the system as it hollows are very low, causing an unusually low oscillation frequency and an associated dip in the mode spectrum. We explicitly demonstrate these effects in the simplest case of spherical symmetry; our results are summarized in Figs. 2 and 4. As is common and effective for collective mode analyses in BECs, we employ the hydrodynamic approach, which models excitations with relatively small, smooth deviations from the equilibrium density of the condensate. (Oscillations of certain low lying modes have been obtained by a variational approach in the literature [49] as well.) The collective eigenmode frequency ω is then related to density fluctuations in space and time via δn(r, t) = δn(r)e−iωt [36, 54]. The eigenvalue equation for the collective modes and their frequencies is given by
更多查看译文
AI 理解论文
溯源树
样例
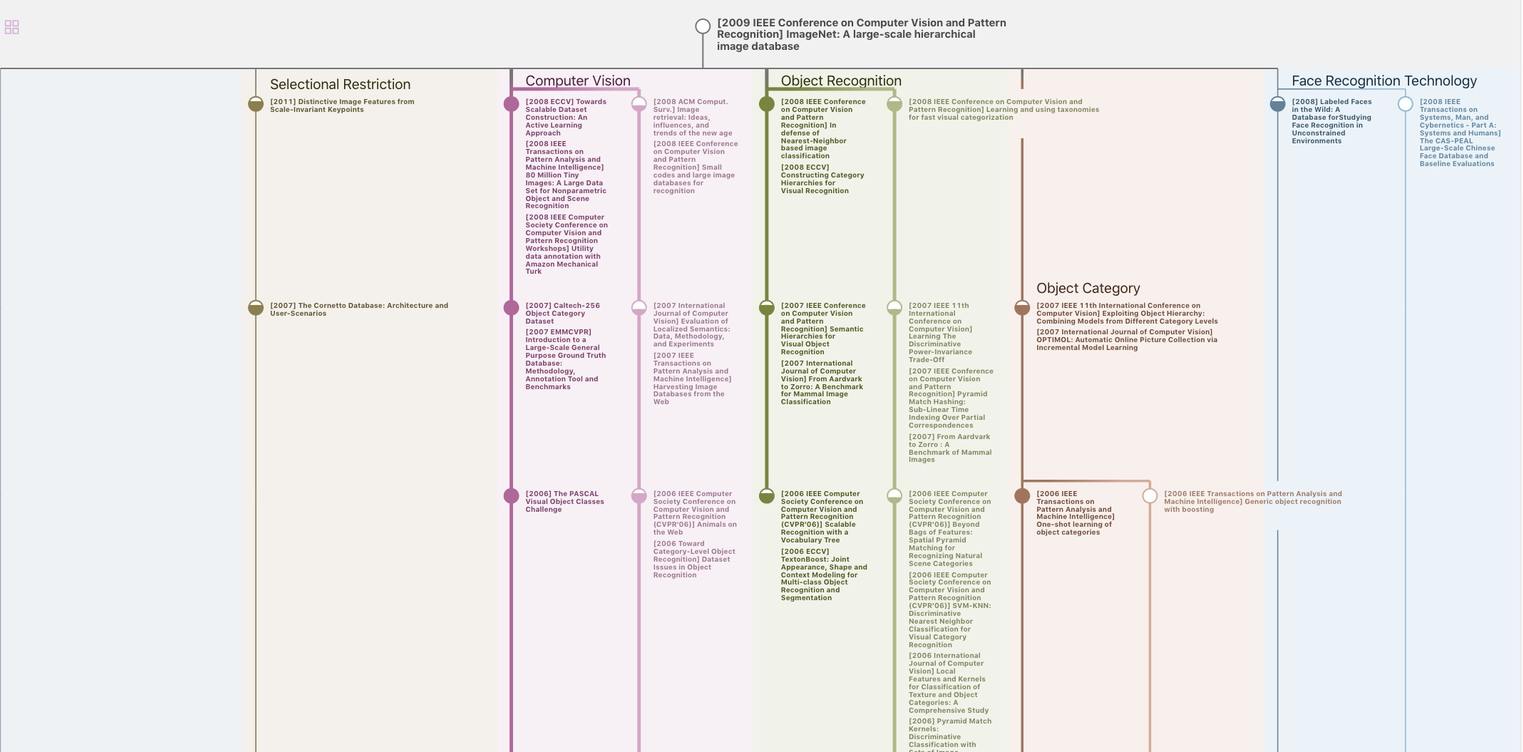
生成溯源树,研究论文发展脉络
Chat Paper
正在生成论文摘要