PEO-imidazole ionic liquid-based electrolyte and the influence of NMBI on dye-sensitized solar cells
Electrochimica Acta(2011)
摘要
Highlights ► Ionic liquid oligomer (PEOImI) is applied as electrolyte for DSSC to improve its efficiency. ► 1-Alkyl-3-methylimidazolium iodide is mixed with PEOImI to form electrolyte. ► The efficiency is further improved by adding N-methylimidazole (NMBI). ► NMBI enhances the Grotthus type diffusion of triiodide. ► A highest energy conversion efficiency of 6.14% has been attached at 100 mW cm −2 . Abstract 1-Oligo(ethylene oxide)-3-methylimidazolium iodide (PEOMImI) was synthesized and applied to dye-sensitized solar cells (DSSCs) by blending it with different 1-alkyl-3-methylimidazolium iodides used as ionic liquid electrolytes. The 1-propyl-3-methylimidazolium iodide (PMII) blend enabled the DSSC to attain a higher solar energy conversion efficiency of 4.52% under a light intensity of 100 mW cm −2 . The addition of N-methylimidazole (NMBI) to the electrolytes increased the conversion efficiency as compared to DSSCs based on NMBI-free electrolytes. The addition of both 1-allyl-3-methylimidazolium iodide (AMII) and NMBI enabled DSSCs to reach their highest solar energy conversion efficiency of 6.14% under a light intensity of 100 mW cm −2 . The ionic conductivity and diffusion coefficient of the triiodide were found to be augmented dramatically after adding NMBI, which leads to an increase in the photocurrent density. The enhancement mechanism of NMBI in the electrolyte was investigated by Raman spectroscopy and differential scanning calorimetry, and it was mainly due to the enhancement of electron exchange in electrolytes. Keywords Ionic liquid Electrolyte NMBI Dye-sensitized solar cell 1 Introduction Dye-sensitized solar cells (DSSCs) have received much attention as potential light-to-electric devices because of their high efficiency and low cost [1] . DSSCs prepared with solvent-based volatile electrolytes have reached conversion efficiencies of 11.04%. However, due to the evaporation and leakage of the liquid electrolyte, the cell performance decays during long-term operation. Room-temperature ionic liquids (RTILs), especially imidazolium salts, are promising candidates to replace volatile solvents because of their chemical and thermal stability, non-volatility, high ionic conductivity, large electrochemical window and good solvent behavior. Much attention has been focused on this topic. However, the high viscosity of RTILs seems to limit charge transport in the electrolyte. To lower the viscosity, Grätzel and his co-workers developed binary ionic liquid electrolytes by mixing ionic liquids (ILs) containing different anions, such as tetracyanoborate (B(CN) 4 ), tricyanomethanide (TCM), dicyanamide (DCA)and thiocyanate (SCN), to accelerate charge transport [2–7] . Peng Wang reported electrolytes prepared by mixing several imidazolium iodides with different alkyl chain lengths [8–10] . Yanagida used 1-dodecyl-3-methylimidazolium iodide, a new ionic liquid crystal, as a hole transport layer [11] ; however, the photocurrent density of the DSSC based on that electrolyte seems to be low due to its high viscosity. Miao Wang from our group investigated electrolytes using ionic liquid oligomers based on poly(ethylene oxide) (PEO; MW = 150, 350, 550 and 750) and imidazolium chloride. PEO exhibits high chemical stability and excellent solvency for salts. Combing PEO with imidazolium can solve the problem of its crystallization below 60 °C. The conversion efficiency of DSSCs with PEO ionic liquids reaches 3.1% at an irradiation of 100 mW cm −2 [13] . Chloride anions lower the concentration of I − ions, which may negatively affect the DSSC. Additives, such as tert-butylpyridine (TBP) and N-methylimidazole (NMBI), were used in the electrolytes based on organic solvents or ionic liquids to increase the photoelectric conversion efficiency. These additives increase the open circuit voltage ( V oc ) with a slight decrease in the photocurrent density due to the influence of additives on the interface between TiO 2 and the electrolyte [12] . However, the influence of additives on mass transport in the electrolyte has not been extensively researched. In our work, 1-oligo(ethylene oxide)-3-methylimidazolium iodide (PEOMImI) was synthesized and mixed with different 1-alkyl-3-methylimidazolium iodides. By incorporating a PEO oligomer with an imidazolium ionic liquid, the conductivity was enhanced primarily by improving cation transport in the PEO segment while the viscosity did not decrease due to the weak interaction of ethylene oxide segment compared to the alkyl chain. After adding NMBI to the electrolytes, the conversion efficiency increased dramatically compared to the electrolytes without NMBI. The performance of NMBI was different from that in the other electrolytes. After NMBI was added to the electrolyte, the photovoltage and the photocurrent increased, and the ionic conductivity and the apparent diffusion coefficient ( D app ) of the triiodide for the corresponding electrolyte increased sharply. The charge transport mechanism in this system is proposed to explain the augmenting of the ionic conductivity and apparent diffusion coefficient. 2 Experiment 2.1 Materials Poly(ethylene glycol) methyl ether (MW = 1000) and N-methylimidazole were purchased from Aldrich Chemical Co. Ltd USA. Iodine (I 2 ) was purchased from Acros USA. Iodoethane, 1-iodopropane, allyl iodide, 1-iodobutane, 1-iodohexane and 1-methylbenzimidazole (NMBI) were purchased form Alfa Aesar UK. N,N-dimethylformamide (DMF) and acetone were purchased from Beijing Chemical Company China (analytic reagent grade). 2.2 Preparation of TiO 2 electrodes and the fabrication of DSSC The nanocrystalline TiO 2 colloid was prepared by the hydrothermal method described elsewhere [14] . Prior to film preparation, 10 wt% polystyrene with a particulate diameter of 40 nm was mixed with a TiO 2 colloidal paste (colloid 40) to increase the porosity of the TiO 2 film. For the preparation of the TiO 2 electrode, the fluorine-doped tin oxide (FTO) glass substrate was first coated with a titanium isopropoxide butanol solution to produce a compact layer of TiO 2 . After sintering at 450 °C for 30 min, the colloid 40 mixture was coated on the electrode. The prepared electrode was then sintered at 450 °C for 30 min. The overall thickness of the TiO 2 film was about 6 μm. After cooling to 80 °C, the TiO 2 electrode was dipped into 0.5 mM cis-di(thiocyanato)-N,N-bis(2,2′-bipyridyl-4, 4′-dicarboxylic acid) ruthenium(III) complex ([RuL 2 (NCS) 2 ]) in absolute ethanol for 24 h. Afterwards, the electrode was rinsed with absolute ethanol and air-dried. A counter electrode is a Pt-sputtered FTO conducting glass. DSSCs were fabricated by injecting the ionic liquid electrolyte into the gap between the dye-sensitized nanocrystalline TiO 2 electrode and the counter electrode clamped with a holder. 2.3 Synthesis and characterization of 1-oligo(ethylene oxide)-3-methylimidazolium iodide 1-Oligo(ethylene oxide)-3-methylimidazolium chloride (PEOImCl) with PEO (MW = 1000) was synthesized according to the previously published method [13] . The formation of PEOImCl was confirmed with the IR spectrum, which showed a new absorption peak at 663 cm −1 that corresponds to the stretching of C–Cl and the disappearance of the strong stretching absorption for –OH at 3400–3550 cm −1 . 1-Oligo(ethylene oxide)-3-methylimidazolium iodide (PEOImI) was prepared as follows. First, PEO-Cl was dissolved in acetone and mixed with an excess of NaI. The mixture was stirred at 80 °C for 3 days under N 2 atmosphere. PEOImI was confirmed by nuclear magnetic resonance (NMR): 1 H NMR (300 MHz, CDCl 3 ) δ 9.88 (s, 1H), 7.74 (s, 1H), 7.42 (s, 1H), 4.58 (s, 2H), 4.03 (s, 3H), 3.60 (br s, 104H) and 3.36 (s, 3H). The other ionic liquids were synthesized according to the literature [15] . All of the ionic liquids were dried under vacuum at 60 °C for 24 h. 2.4 Measurements of current–voltage characteristics The cells were illuminated by a Newport solar simulator (69911) under AM1.5 (100 mW cm −2 ) with an active area of 0.25 cm 2 for the photovoltaic measurements. A PAR potentiostat (EG & G Princeton Applied Research, model 273) was employed to record the photovoltaic performances of the cells. 2.5 Measurement of the apparent diffusion coefficient of I 3 − The apparent diffusion coefficient ( D app ) of the triiodide in the electrolyte was measured with a Solartron SI 1287 electrochemical instrument. The sample was placed in a poly(tetrafluoroethylene) spacer ring compressed by two Pt electrodes. The scan rate was 20 mV s −1 . All of the measurements were performed at room temperature. 2.6 Ionic conductivity of the ionic liquid electrolytes The ionic conductivity of the ionic liquid electrolytes was measured using a Solartron SI 1287 electrochemical interface and a Solartron 1255B frequency response analyzer. The polymer electrolytes were placed in a poly(tetrafluoroethylene) spacer ring compressed by two stainless steel electrodes and sealed in a testing cell. 2.7 Raman spectroscopy The Raman measurements were acquired using spectrometer RFS 100/S (Brucker, Karlsruhe, Germany) with a diode-pumped Nd:YAG laser at an operating wavelength of 1064 nm. The measurements were performed using the 180° angle scattering geometry with 50 scans and a laser power of 300 mW at the sample location. 2.8 Differential scanning calorimetry measurements The differential scanning calorimetry (DSC) studies were performed on a Mettler-822e. The 10 mg sample was measured at a heating rate of 10 °C min −1 in the temperature range of −60 to 60 °C. 3 Results and discussion 3.1 Ionic conductivity and the apparent diffusion coefficient of triiodides in the ionic liquid oligomer electrolytes The ionic conductivity of the ionic liquid electrolyte was measured by electrochemical impedance spectroscopy (EIS), and the bulk resistance was determined at 1000 Hz. The conductivity of the electrolyte was obtained using the following equation: (1) σ = L R S where L is the distance between the two stainless steel electrodes and is regarded as the thickness of the ionic liquid electrolyte, R is the bulk resistance and S is the cross-sectional area of the stainless steel electrode. Electrolytes A to F are composed of 0.1 M I 2 in different kinds of 1-alkyl-3-methylimidazolium (i.e., 1,3-dimethylimidazolium (DMII), 1-ethyl-3-methylimidazolium iodide (EMII), 1-propyl-3-methylimidazolium iodide (PMII), 1-allyl-3-methylimidazolium iodide (AMII), 1-butyl-3-methylimidazolium iodide (BMII) and 1-hexyl-3-methylimidazolium iodide (HMII), respectively) and with PEOImI (1:1 volume ratio). NMBI is added to each sample. The ionic conductivities for electrolytes A to F with and without the addition of NMBI are shown in Fig. 1 a and listed in Table 1 . Before adding NMBI, the ambient ionic conductivity of the electrolyte increased with the initial increases in alkyl chain length of the 1-alkyl-3-methylimidazolium iodides but then decreased with further increases in the alkyl chain length. The peak value of the ionic conductivity was 1.78 × 10 −3 S cm −1 , which was obtained for electrolyte C composed of MPII. This result may be explained according to ion dissociation and transportation. When the alkyl chain length is short, the ion pair is difficult to dissociate because the interaction between ion pairs is too strong. The ion diffusion rate slows with increasing alkyl chain length because of van der Waals forces [16] . After adding NMBI, the ionic conductivity significantly increases for each electrolyte and reaches the highest value 10.67 × 10 −3 S cm −1 for electrolyte A containing DMII. This value is approximately one order larger than that without NMBI. The introduction of NMBI plays an important role in the increase of ionic conductivity, which might be attributed to the weakened interaction between the ion pairs benefiting the charge transportation, which will be discussed later in this paper. The measured ionic conductivity includes contributions from both anions and cations in the electrolyte, but the D app of the triiodide would more directly influence the performance of the DSSC. Therefore, the D app of the triiodide in our ionic liquid electrolyte was obtained by measuring the steady-state current with two Pt electrodes. J lim depends on the D app and is given by the following equation [17] : (2) J lim = 2 n F D a p p C 0 d , where n is the electron number of each molecule ( n equals to 2), F is the Faraday constant, C is the bulk concentration of the triiodide and d is the thickness of the electrolyte membrane. Table 2 lists the D app values of the triiodide in electrolytes A to F, with and without NMBI. Fig. 1 b shows that the change of D app (I 3 − ) is similarly regulated as is the ionic conductivity. In summary, we conclude that the alkyl chain lengths that are either too short or too long are not helpful for ionic conductivity and D app (I 3 − ). Electrolyte A without NMBI shows a low D app for the triiodide. This result might be attributed to DMII being solid at room temperature and not being easily dissolved in the PEOImI ionic liquid. The electrolytes containing MPII and AMII reached the highest value. The introduction of NMBI significantly increases the ionic conductivity and D app (I 3 − ), which should participate in ion dissociation and charge transportation. 3.2 Roles of the additive in the electrolyte NMBI as an additive in electrolytes has generally been reported to increase the V oc of DSSC by adsorbing onto the surface of TiO 2 . We found that this additive has a positive effect on ionic conductivity and D app (I 3 − ) and investigated the possible mechanism by which NMBI works in electrolyte D, which is based on PEOImI and AMII. The melting point ( T m ) of an electrolyte has an influence on charge transport and the application environment. The T m of electrolyte D decreased after adding NMBI. Fig. 2 shows the DSC curves of electrolyte D, with and without NMBI. The peak at −42 °C was assigned to the glass transition temperature ( T g ) of the ethylene oxide (EO) segment. The curves show nearly the same value, indicating that the introduction of NMBI has no influence on the T g of the EO segment. Another peak at about −20 °C was assigned to the T m of the electrolyte. After adding NMBI, the T m decreased from −17.0 °C to −23.5 °C. These results indicate that NMBI can weaken the Coulomb force between the cation and the anion, meaning that it is easier for ion dissociation. In the ionic liquid complex system, charge transfer depends on physical diffusion and electron-exchange diffusion due to the high viscosity. The formation of I 3 − and polyiodides is essential for chemical diffusion, generally termed as a Grotthus-type mechanism [18] . Fig. 3 shows the Raman spectra of electrolyte D, with and without NMBI. A Raman band at 113 cm −1 was assigned to the symmetric stretch of I 3 − , and another band at about 146 cm −1 was assigned to the vibration mode of the polyiodides. By adding NMBI to the electrolyte, the band intensities of I 3 − and the polyiodides decrease, and the band position of the polyiodides shifts from 145.7 cm −1 to 147.6 cm −1 , which indicates that the concentrations of I 3 − and polyiodides decrease and the chemical bond I–I is weakened in the polyiodides. According to [19] , NMBI reacts with I 3 − in a similar way as TBP: (3) NMBI + I 3 − ⇋ NMBII 2 + I − (4) 2NMBI + I 3 − ⇋ (NMBI) 2 I + + 2I − This reaction may be the reason that the concentration of I 3 − decreases, and it would cause the concentration of I − to increase, which is helpful for the regeneration of the dye. Polyiodides may also show similar reactions with NMBI. Polymeric chains of ethylene oxide have the ability to transport cations. Thus, the formation of (NMBI) 2 I + would increase the concentration of ions and enhance the charge transport together with the collaborative effect of the EO segment. The red shift of the I n − band implies that the chemical bond I–I of I n − is weakened due to the enhancement of the donor–acceptor interaction between NMBI and I n − . This weakening might be attributed to π–π interactions between NMBI and the imidazolium cations. In the Grothuss-type charge transfer mechanism, electron hopping and polyiodide bond exchange are coupled, favoring the effective conductance of the ionic liquid electrolyte. The weakened chemical bond of I-I facilitates bond exchange, thus enhancing the diffusion coefficient of I n − . The progress of charge transportation is described in Fig. 4 . The formation of (NMBI) 2 I + and the weakening of I–I suggest that NMBI has a positive effect on ion dissociation and charge transportation, which makes the ionic conductivity and D app (I 3 − ) increase. 3.3 Photovoltaic performance of DSSCs Fig. 5 shows the current–voltage curves of a DSSC employing the electrolytes based on different 1-alkyl-3-methylimidazolium iodides mixings in PEOImI without NMBI at an irradiation of 100 mW cm −2 . Table 3 lists the photovoltaic parameters obtained from Fig. 5 and the apparent diffusion coefficient ( D app ) of I 3 − . Fig. 6 and Table 4 show the corresponding parameters with the addition of NMBI. The short circuit current ( J sc ), open circuit voltage ( V oc ) and fill factor (ff) of the DSSCs based on electrolytes containing NMBI are all higher than those of DSSCs based on the corresponding electrolytes without NMBI. Without the addition of NMBI, the short circuit current first increases and then decreases with the increase in alkyl chain length. The change trend of J sc with alkyl chain length is related to that of D app for I 3 − , which illustrates that the diffusion of I 3 − is the current limiting process. Therefore, in these electrolytes, augmenting the D app of I 3 − is an effective way to improve the J sc . In the presence of NMBI, J sc increases for all of the electrolytes, which is mainly caused by the increase in the D app of I 3 − . To further confirm the effect of the D app for I 3 − on J sc , we measured the J sc of DSSCs for different light intensities. Fig. 7 shows the dependence of J sc on the incident light intensity, which provides information on the mass transport limitation in the electrolytes, with and without NMBI. Before adding NMBI, the J sc values of DSSCs based on electrolytes A and B increased proportionally with the light intensity up to 100 mW cm −2 . These results imply that J sc is not limited by the diffusion of I 3 − in electrolytes A and B until the light intensity reaches 100 mW cm −2 . However, in the case of DSSCs based on electrolytes C–F, J sc increased linearly with the light intensity at the beginning and then increased slowly to a saturation above 60 mW cm −2 . This result indicates the limitation of J sc at high light intensities. After adding NMBI, all of the J sc values obtained in the DSSCs based on electrolytes A to F increased proportionally with a light intensity of up to 100 mW cm −2 . These results imply that the diffusion of the triiodide does not limit the overall photocurrent density of the cell, even during high-intensity irradiation, due to the much larger D app of I 3 − in the electrolyte containing NMBI compared to the electrolyte without NMBI. Before adding NMBI, V oc first increases and then decreases with increasing alkyl chain lengths of the 1-alkyl-3-methylimidazolium iodides. The maximum V oc is obtained in the case of HMII. The larger V oc is mainly due to the suppression of the dark current, which arises from triiodide reduction at the nanocrystalline TiO 2 /electrolyte interface by electrons within the conduction band of nanocrystalline TiO 2 . Imidazolium cations absorbed at the TiO 2 surface play an important role in blocking the surface states, which act as the mediators of charge transfer from the conduction band of TiO 2 to the triiodide. A decrease in the dark current density with the increase in alkyl chain length is observed in Fig. 4 . With the increase in the alkyl chain length of the imidazolium cations, the recombination of electrons in the conduction band of electrodes with I 3 − in the electrolyte appears difficult. After adding NMBI, V oc increases compared to the electrolyte without NMBI. V oc slightly changes due to the increase in alkyl chain length. This change is attributed to the absorption of NMBI at the TiO 2 surface, suppressing the surface recombination via passivation of the surface states. NMBI shows a similar effect as seen for TBP [20] . The highest light-to-electron efficiency at 100 mW cm −2 was obtained with the DSSC based on electrolyte D with AMII and NMBI. The corresponding photovoltaic parameters of this cell are 12.50 mA cm −2 ( J sc ), 680 mV ( V oc ), 0.72 (ff) and 6.14% ( η ). 4 Conclusions PEOImI was synthesized by incorporating imidazolium ionic liquid with PEO oligomers. By mixing PEO oligomers with different kinds of 1-alkyl-3-methylimidazolium iodides, a series of ionic liquid electrolytes was prepared. High ionic conductivity and a large apparent diffusion coefficient for the triiodide were exhibited in these electrolytes. We investigated the influence of the alkyl chain length of 1-alkyl-3-methylimidazolium iodides on the ionic conductivity and apparent diffusion coefficient due to the interaction between cations and anions. The ionic conductivity and apparent diffusion coefficient were improved by adding NMBI, which contributes to the enhancement of electron exchange of I − /I 3 − redox species as confirmed by DSC and Raman spectra. The addition of NMBI improves V oc and J sc by increasing the ionic conductivity and apparent diffusion coefficient. The highest conversion efficiency (6.14%) under irradiation of 100 mW cm −2 was achieved for the DSSC employing the electrolyte containing AMII. Acknowledgements This work was supported by the National Research Fund for Fundamental Key Project (2006CB202605), the High-Tech Research and Development of China Program (2007AA05Z439) and the Nation Natural Science Foundation of China ( 50973116 ). References [1] B. O’Regan M. Grätzel Nature 353 1991 737 [2] D. Kuang P. Wang S. Ito S.M. Zakeeruddin M. Grätzel J. Am. Chem. Soc. 128 2006 7732 [3] D. Kuang C. Klein Z. Zhang S. Ito J.-E. Moser S.M. Zakeeruddin M. Grätzel Small 3 2007 2094 [4] Y. Bai Y. Cao J. Zhang M. Wang R. Li P. Wang S.M. Zakeeruddin M. Grätzel Nat. Mater. 7 2008 626 [5] P. Wang B. Wenger R. Humphry-Baker J.-E. Moser J. Teuscher W. Kantlehner J. Mezger E.V. Stoyanov S.M. Zakeeruddin M. Grätzel J. Am. Chem. Soc. 127 2005 6850 [6] P. Wang S.M. Zakeeruddin J.-E. Moser M. Grätzel J. Phys. Chem. B 107 2003 13280 [7] P. Wang S.M. Zakeeruddin R. Humphry-Baker M. Grätzel Chem. Mater. 16 2004 2694 [8] Y. Bai Y.M. Cao J. Zhang M. Wang R.Z. Li P. Wang S.M. Zakeeruddin M. Grätzel Nat. Mater. 7 2008 626 [9] Y.M. Cao J. Zhang Y. Bai R.Z. Li S.M. Zakeeruddin M. Grätzel P. Wang J. Phys. Chem. C 112 2008 13775 [10] D. Shi N. Pootrakulchote R.Z. Li J. Guo Y. Wang S.M. Zakeeruddin M. Grätzel P. Wang J. Phys. Chem. C 112 2008 17046 [11] N. Yamanaka R. Kawano W. Kubo T. Kitamura Y. Wada M. Watanabe S. Yanagida Chem. Commun. 2005 740 [12] H. Kusama Y. Konishi H. Sugihara H. Arakawa Solar Energy Mater. Solar Cells 2 2003 167 [13] M. Wang X. Xiao X. Zhou X. Li Y. Lin Solar Energy Mater. Solar Cells 91 2007 785 [14] Y.F. Zhou W.C. Xiang S. Chen S.B. Fang X.W. Zhou J.B. Zhang Y. Lin Electrochim. Acta 54 2009 6645 [15] P. Bonhote A.P. Dias N. Papageorgiou K. Kalyanasundaram M. Grätzel Inorg. Chem. 35 1996 1168 [16] W. Kubo T. Kitamura K. Hanabusa Y. Wada S. Yanagida Chem. Commun. 2002 374 [17] Z. Kebede S.E. Lindquist Solar Energy Mater. Solar Cells 57 1999 259 [18] P. Wang S.M. Zakeeruddin I. Exnar M. Grätzel Chem. Commun. 2002 2972 [19] G. Boschloo L. Haggman A. Hagfeldt J. Phys. Chem. B 110 2006 13144 [20] K. Hara Y. Dan-oh C. Kasada Y. Ohga A. Shinpo S. Suga K. Sayama H. Arakawa Langmuir 2004 4205
更多查看译文
关键词
Ionic liquid,Electrolyte,NMBI,Dye-sensitized solar cell
AI 理解论文
溯源树
样例
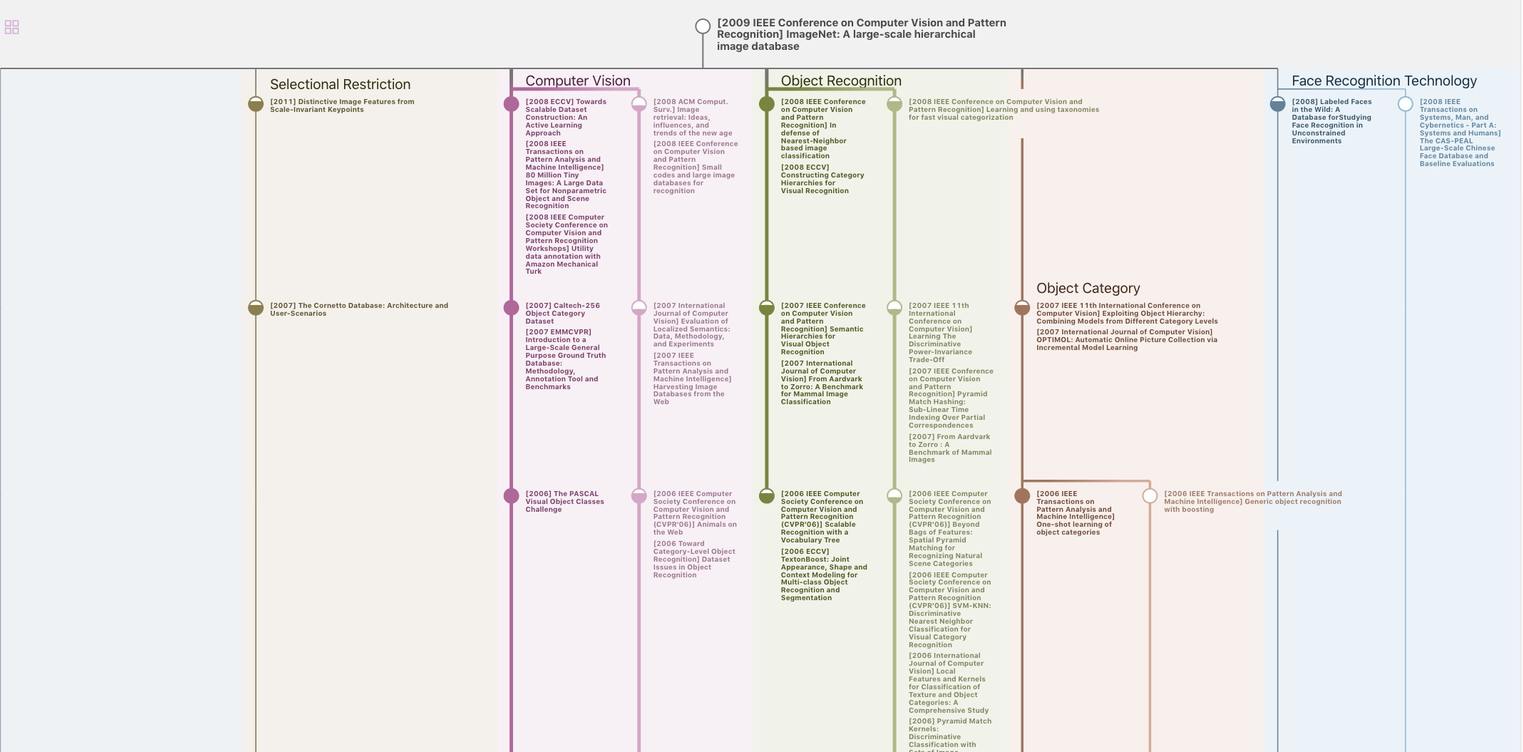
生成溯源树,研究论文发展脉络
Chat Paper
正在生成论文摘要