Magnetomechanical properties of epoxy-bonded Sm1−xNdxFe1.55 (0≤x≤0.56) pseudo-1–3 magnetostrictive particulate composites
Journal of Alloys and Compounds(2011)
摘要
Research highlights ► We fabricate pseudo-1−3 magnetostrictive particulate composites by embedding and aligning light rare earth (Sm and Nd)-based magnetostrictive Sm 1 –x Nd x Fe 1.55 ( x = 0–0.56) particles in a passive epoxy matrix. ► We measure the quasistatic magnetomechanical properties of the composites and compare the measured results with the monolithic alloys for various x . ► The composites exhibit similar qualitative trends in properties with the alloys for all x . ► The Sm 0.92 Nd 0.08 Fe 1.55 composite shows the best negative magnetomechanical properties as a result of the magnetocrystalline anisotropy compensation between Sm 3+ and Nd 3+ ions in the Sm 0.92 Nd 0.08 Fe 1.55 alloy. Abstract Pseudo-1–3 magnetostrictive particulate composites consisting of light rare earth (Sm and Nd)-based magnetostrictive Sm 1− x Nd x Fe 1.55 particles with the Nd content x of 0–0.56 and randomly distributed sizes of 10–180 μm embedded and aligned in a passive epoxy matrix are fabricated using the particulate volume fraction of 0.5. The quasistatic magnetomechanical properties of the composites are investigated and the results are compared with their monolithic alloys for various x . The composites exhibit similar qualitative trends in properties with the alloys for all x . The Sm 0.92 Nd 0.08 Fe 1.55 composite shows a large unsaturated magnetostriction λ of −530 ppm at 500 kA/m and a high piezomagnetic coefficient d 33 of −2.0 nm/A at 100 kA/m as a result of the magnetocrystalline anisotropy compensation between Sm 3+ and Nd 3+ ions in the Sm 0.92 Nd 0.08 Fe 1.55 alloy. Keywords Light rare earths Magnetocrystalline anisotropy Magnetomechanical properties Negative magnetostriction Magnetostrictive composites Sm 1− x Nd x Fe 1.55 alloys 1 Introduction Pseudobinary RR′Fe 2 Laves alloys (R, R′ rare earths, where R ≠ R′) have received much attention in recent years for magnetostrictive transducer and actuator applications because of their potentially high magnetostrictions and low magnetocrystalline anisotropy at room temperature [1,2] . To reduce eddy-current losses and mechanical brittleness intrinsic in the alloys, special attention has been paid to develop epoxy-bonded magnetostrictive particulate composites [3–8] . In fact, composites with pseudo-1–3 configuration (i.e., aligning magnetostrictive particles in a passive polymer matrix) generally demonstrate higher magnetomechanical properties than those with 0–3 configuration (i.e., dispersing particles in the matrix) due to their anisotropic nature [6,7] . Moreover, there exists an optimal particulate volume fraction of 0.5 in the pseudo-1–3 composites by taking the tradeoff between the maximization of magnetomechanical properties and the minimization of material cost into consideration [8] . Among the available RR′Fe 2 Laves alloys, Tb 0.3 Dy 0.7 Fe 1.92 (Terfenol-D) and Sm 0.88 Dy 0.12 Fe 1.93 (Samfenol-D) have been widely investigated and shown to possess giant positive and negative magnetostrictions, respectively [9,10] . Since the main functional components of Terfenol-D and Samfenol-D involve scanty and expensive heavy rare earths Tb and Dy, it will be a great benefit to applications if these heavy rare earths in the ordinary Terfenol-D and Samfenol-D compositions can be substituted by abundant and cost-effective light rare earths and maintain competitive properties. To date, literature reports have been mainly on light rare earth-substituted positive magnetostrictive alloy systems. Accordingly, several good candidates have been prepared by substituting Pr or Nd for Tb and/or Dy in Terfenol-D. These include: (Tb 0.3 Dy 0.7 ) 1− x Pr x Fe 1.55 (0 ≤ x ≤ 0.4) [11] , Tb 0.2 Dy 0.8− x Pr x (Fe 0.9 B 0.1 ) 1.93 (0 ≤ x ≤ 0.4) [12] , Tb x Nd 1− x Fe 1.9 (0 ≤ x ≤ 0.8) [13] , etc. For the equally important negative magnetostrictive alloy systems, reports are relatively limited. Recently, we have synthesized light rare earth-based Sm 1− x Nd x Fe 1.55 (0 ≤ x ≤ 0.56) Laves alloys showing high negative magnetostrictions [14] . Nd is considered as a good candidate for partial substitution in stable SmFe 2 because NdFe 2 has opposite anisotropy and a small positive magnetostriction [15–17] . In this work, we aim to extend our work on Sm 1− x Nd x Fe 1.55 (0 ≤ x ≤ 0.56) Laves alloys to fabricate epoxy-bonded Sm 1− x Nd x Fe 1.55 (0 ≤ x ≤ 0.56) pseudo-1–3 magnetostrictive particulate composites and investigate their quasistatic magnetomechanical properties at a frequency of 0.05 Hz. Comparison of the measured properties between the composites and the alloys is made on the same function of Nd content x in order to obtain a relatively complete picture on the Sm 1− x Nd x Fe 1.55 (0 ≤ x ≤ 0.56) system. 2 Experimental details Polycrystalline Sm 1− x Nd x Fe 1.55 (0 ≤ x ≤ 0.56) alloys with 10 different Nd contents x of 0, 0.08, 0.12, 0.16, 0.20, 0.24, 0.32, 0.40, 0.48, and 0.56 were prepared by arc-melting the constituent metals in a high-purity argon atmosphere [14] . The constituent metals were commercially acquired and their purities were 99.9% for Sm and Nd and 99.8% for Fe. As the vapor pressure of Sm was rather high during arc-melting, an extra 3 wt% Sm was adopted to compensate the volatilization of Sm during the arc-melting process. The ingots were sealed in a quartz tube and homogenized at 873 K for 7 days in a high-purity argon atmosphere. The homogenized ingots were ground into particles with randomly distributed sizes. Two different sieves were used to eliminate any particles smaller than 10 μm and larger than 180 μm, giving a preferred particulate size range of 10–180 μm. X-ray diffractrometry with CuKα radiation was applied to the homogenized particles at room temperature in a Riguku D/max-2500pc diffractrometer equipped with a graphite monochromator. The X-ray diffraction (XRD) analysis indicated that the alloys with the Nd content x varying from 0 to 0.48 contain mainly cubic Laves phase, with minor rare-earth phases. For the alloys having an elevated x of 0.56, the cubic Laves phase coexisted with a small amount of (Sm, Nd)Fe 3 because NdFe 2 can only be formed at high pressures. In fact, it was reported that the lattice parameter a increases with increasing x , while the Curie temperature T c trends to the opposite way [14] . To fabricate the epoxy-bonded Sm 1− x Nd x Fe 1.55 (0 ≤ x ≤ 0.56) pseudo-1–3 composites, predetermined quantities of Sm 1− x Nd x Fe 1.55 (0 ≤ x ≤ 0.56) particles ground from the homogenized ingots were homogeneously mixed with Araldite LY5210/HY2954 epoxy in plastic molds with a rectangular cavity of dimensions 7 mm × 7 mm × 28 mm. The mixed slurries were degassed under vacuum for 30 min to eliminate air bubbles. The molds were then sealed to prevent particles from migrating out once they were placed between a pair of Nd–Fe–B permanent magnets. These magnets produced a uniform dc magnetic field of about 150 kA/m along the longitudinal direction of the molds, causing the enclosed particles to align with the magnetic flux lines and producing particulate chains similar to pseudo-fiber composites or, in general, pseudo-1–3 particulate composites [4,8] . After the particles had been lengthwise-aligned in the molds, the entire mold-magnet assemblies were placed in a temperature-controlled oven at 80 °C for 10 h to ensure full cure of the epoxy and to impart an average axial residual compressive stress of about 3 MPa to the particulate chains through the thermal shrinkage of the epoxy in the composites [18] . This built-in residual compressive stress was shown to be effective in creating a preferred non-180° domain state in the as-prepared composites even though no external stress was used [4,5] . The effect is similar to the case of applying an external prestress to assert an initial non-180° domain state in monolithic Terfenol-D [19] . After being demolded, the particulate (Sm 1− x Nd x Fe 1.55 ) volume fraction of the composites was determined to be 0.5 based on Archimedes’ principle and rule-of-mixture formulation for density expressed below: (1) ρ c = v f ρ a + ( 1 − v f ) ρ e , where ρ c , ρ a , and ρ e are the densities of the composite, alloy (=8300 kg/m 3 ), and epoxy (1097 kg/m 3 ), respectively; and v f is the particulate volume fraction of the composites. An in-house automated magnetostrictive measurement system was used to measure the quasistatic magnetomechanical properties of the composites and alloys in the longitudinal direction at room temperature and with zero stress bias [4] . These included the magnetization–magnetic field ( M – H ) curves and the magnetostriction–magnetic field ( λ – H ) curves. The M – H and λ – H curves were acquired by energizing a water-cooled electromagnet to provide a cyclic magnetic field ( H ) with a maximum amplitude of 500 kA/m at a quasistatic frequency of 0.05 Hz and then measuring the corresponding magnetic flux density ( B ) and magnetostriction ( λ ) using a search coil wrapped around the samples and a strain gauge attached to the center of the samples, respectively. It is noted in the measurement that the longitudinal direction of the samples was aligned parallel to the direction of H so that the measured B and λ were essentially the longitudinal values. The magnetization ( M ) was calculated using (2) M = B μ 0 − H , where μ 0 = 4 π × 10 −7 H/m is the permeability of free space. The piezomagnetic coefficient ( d 33 ) was obtained from the λ – H plots using (3) d 33 = ∂ λ ∂ H 3 Results and discussion Fig. 1 (a) shows the magnetization–magnetic field (4 πM – H ) curve of the epoxy-bonded Sm 0.6 Nd 0.4 Fe 1.55 composite, while Fig. 1 (b) plots the maximum magnetization (4 πM max ) of the Sm 1− x Nd x Fe 1.55 (0 ≤ x ≤ 0.56) composites and alloys as a function of the Nd content x . It is noted from Fig. 1 (a) that the saturation magnetization state is not obtained at the maximum available H of 500 kA/m. Hence, the value of 4 πM max appeared in Fig. 1 (b) corresponds to that of 4 πM at the maximum available H of 500 kA/m in Fig. 1 (a). Moreover, since 4 πM max of the composites increases slowly with increasing x in Fig. 1 (b), only the 4 πM – H curve of the Sm 0.6 Nd 0.4 Fe 1.55 composite is included in Fig. 1 (a). It is clear that the Sm 0.6 Nd 0.4 Fe 1.55 composite, which is similar to other composites with different x (not shown), exhibits soft magnetic characteristics. For comparison, 4 πM max of the alloys demonstrates a similar quantitative increasing trend with the composites for all x . As the composites with different x have the same particulate volume fraction of 0.5, we believe that the increasing trend observed in the composites is mainly due to the intrinsic magnetic properties of the alloys governed by the larger 4 f moment of Nd 3+ ion compared to the Sm 3+ ion [17] . The magnetostriction–magnetic field ( λ – H ) curves of the epoxy-bonded Sm 1− x Nd x Fe 1.55 (0 ≤ x ≤ 0.56) composites are shown in Fig. 2 (a) , while the dependence of the Nd content x on maximum magnetostriction ( λ max ) (i.e., the value of λ at H = 500 kA/m) for the composites and the alloys is plotted in Fig. 2 (b). From Fig. 2 (a), the saturation magnetostriction state is not achieved at the maximally applied H of 500 kA/m. This reflects the existence of relatively high magnetocrystalline anisotropy in the Sm 1− x Nd x Fe 1.55 (0 ≤ x ≤ 0.56) alloys and their composites. From Fig. 2 (b), λ max increases initially, reaches its maximum at x = 0.08, and then decreases, with increasing x . This indicates that the small substitution of Nd for Sm in SmFe 2 can lead to a reduction in the magnetocrystalline anisotropy of SmFe 2 and hence an enhancement in λ max [14] . However, the enhancement of λ max in the composites is not as obvious as that in the alloys. Among the various composites, the Sm 0.92 Nd 0.08 Fe 1.55 one exhibits the largest λ max of −530 ppm because of the compensation of magnetocrystalline anisotropy between Sm 3+ and Nd 3+ ions. Due to the fact that the SmFe 2 alloy carries a negative magnetostriction sign in opposition to the positive magnetostriction sign carried by the NdFe 2 alloy, there is a general reduction in λ max for x in excess of 0.08 [14] . In general, the composites show a similar quantitative trend in λ max , but with smaller values than the alloys for all x . This is a result of the reduced particulate volume fraction to 0.5 in the composites compared to unity in the alloys. Fig. 3 shows the piezomagnetic coefficient–magnetic field ( d 33 – H ) curves of the epoxy-bonded Sm 1− x Nd x Fe 1.55 (0 ≤ x ≤ 0.56) composites and the dependence of the Nd content x on maximum piezomagnetic coefficient ( d 33max ) for the composites and the alloys. The d 33 – H curves in Fig. 3 (a) for the composites display similar quantitative increasing and decreasing trends, with the maximum d 33 of about −2 nm/A at 100 kA/m for the Sm 0.92 Nd 0.08 Fe 1.55 composite. The initial increase in d 33 with the increase in H can be attributed to the increase in non-180° domain-wall motion. The H at which d 33 is maximized indicates the actuation of the composites in the “burst region” of the λ – H curves ( Fig. 2 (a)) where the non-180° domain-wall motion is maximum. Above this critical H , the domain saturation occurs, resulting in the decrease in d 33 value. As shown in Fig. 3 (b), the composites show a similar quantitative trend in d 33max , but also with smaller values than the alloys for all x owing to the reduced particulate volume fraction to 0.5. To further understand the magnetization process of Sm 0.92 Nd 0.08 Fe 1.55 composite and alloy, the dependence of λ / λ max on M / M max is shown in Fig. 4 . The values of λ max and M max are obtained at H = 500 kA/m from Fig. 2 (b) and Fig. 1 (b), respectively. It is observed that the values of λ / λ max of the composite are generally larger than those of the alloy. Since the 180° domain-wall motion results in changes in M without accompanying λ , while the non-180° domain-wall motion leads to changes in λ rather than those in M. Therefore, it is believed that the enhanced λ / λ max – M / M max response in the composite compared to the alloy originates from the increased non-180° domain-wall motion in the composite during the magnetization process. Moreover, this increased non-180° domain-wall motion results from the axial residual compressive stress developed through the cure of the epoxy matrix in the composite as stated in Section 1 [4,5] . 4 Conclusion We have fabricated pseudo-1–3 magnetostrictive particulate composites of 0.5 particulate volume fraction by embedding and aligning light rare earth (Sm and Nd)-based magnetostrictive Sm 1− x Nd x Fe 1.55 particles with the Nd content x of 0–0.56 and randomly distributed sizes of 10–180 μm in a passive epoxy matrix. Investigations into the dependence of their quasistatic magnetomechanical properties as function of the magnetic field ( H ) and the Nd content x have shown similar qualitative trends with their alloys. The Sm 0.92 Nd 0.08 Fe 1.55 composite has demonstrated the largest unsaturated magnetostriction ( λ max ) of −530 ppm at 500 kA/m and the highest piezomagnetic coefficient ( d 33 ) of −2 nm/A at 100 kA/m, both at room temperature. These attractive magnetomechanical properties make the composite interesting for practical applications. Acknowledgments This work was supported by the Hong Kong Research Grants Council of the HKSAR Government ( PolyU 5266/08E ), T he Hong Kong Polytechnic University ( G-U741 ), the National Natural Science Foundation of China ( 50831006 and 50571098 ), and the National Basic Research Program ( 2010CB934603 ) of the Ministry of Science and Technology of China. References [1] A.E. Clark E.P. Wohlfarth Ferromagnetic Materials vol. 1 1980 North-Holland Amsterdam 531 [2] H.T. Savage R. Abbundi A.E. Clark O.D. McMasters J. Magn. Magn. Mater. 15 1980 609 [3] N. Nersessian S.W. Or G.P. Carman J. Magn. Magn. Mater. 263 2003 101 [4] S.W. Or N. Nersessian G.P. Carman IEEE Trans. Magn. 40 2004 71 [5] S.W. Or T.L. Li H.L.W. Chan J. Appl. Phys. 97 2005 10M308 [6] L. Sandlund M. Fahlander T. Cedell A.E. Clark J.B. Restorff J. Appl. Phys. 75 1994 5656 [7] T.A. Duenas G.P. Carman J. Appl. Phys. 87 2000 4696 [8] T.A. Duenas G.P. Carman J. Appl. Phys. 90 2001 2433 [9] A.E. Clark AIP Conf. Proc. 18 1974 1015 [10] R. Abbundi A.E. Clark O.D. McMasters J. Appl. Phys. 53 1982 2664 [11] X.K. Lv S.W. Or W. Liu X.H. Liu Z.D. Zhang J. Alloys Compd. 476 2009 24 [12] W.J. Ren Z.D. Zhang X.P. Song X.G. Zhao X.M. Jin Appl. Phys. Lett. 82 2003 2664 [13] Y.G. Shi S.L. Tang Y.J. Huang L.Y. Lv Y.W. Du Appl. Phys. Lett. 90 2007 142515 [14] F. Yang W. Liu X.K. Lv B. Li S.Q. Li J. Li Z.D. Zhang J. Magn. Magn. Mater. 322 2010 2095 [15] V. Hari Babu G. Markandeyulu A. Subrahmanyam Appl. Phys. Lett. 90 2007 252513 [16] B.W. Wang W.J. Lee J.S. Song B.K. Min Y.M. Hao J. Appl. Phys. 91 2002 9246 [17] F.E. Pinkerton J.F. Herbst T.W. Capehart M.S. Meyer W.A. Fellberg J. Appl. Phys. 85 1999 1654 [18] T.A. Duenas, Giant magnetostrictive composites, Ph.D. dissertation, Univ. California, Los Angeles, 2001. [19] G. Engdahl Handbook of Giant Magnetostrictive Materials 2000 Academic San Diego, CA
更多查看译文
关键词
Light rare earths,Magnetocrystalline anisotropy,Magnetomechanical properties,Negative magnetostriction,Magnetostrictive composites,Sm1−xNdxFe1.55 alloys
AI 理解论文
溯源树
样例
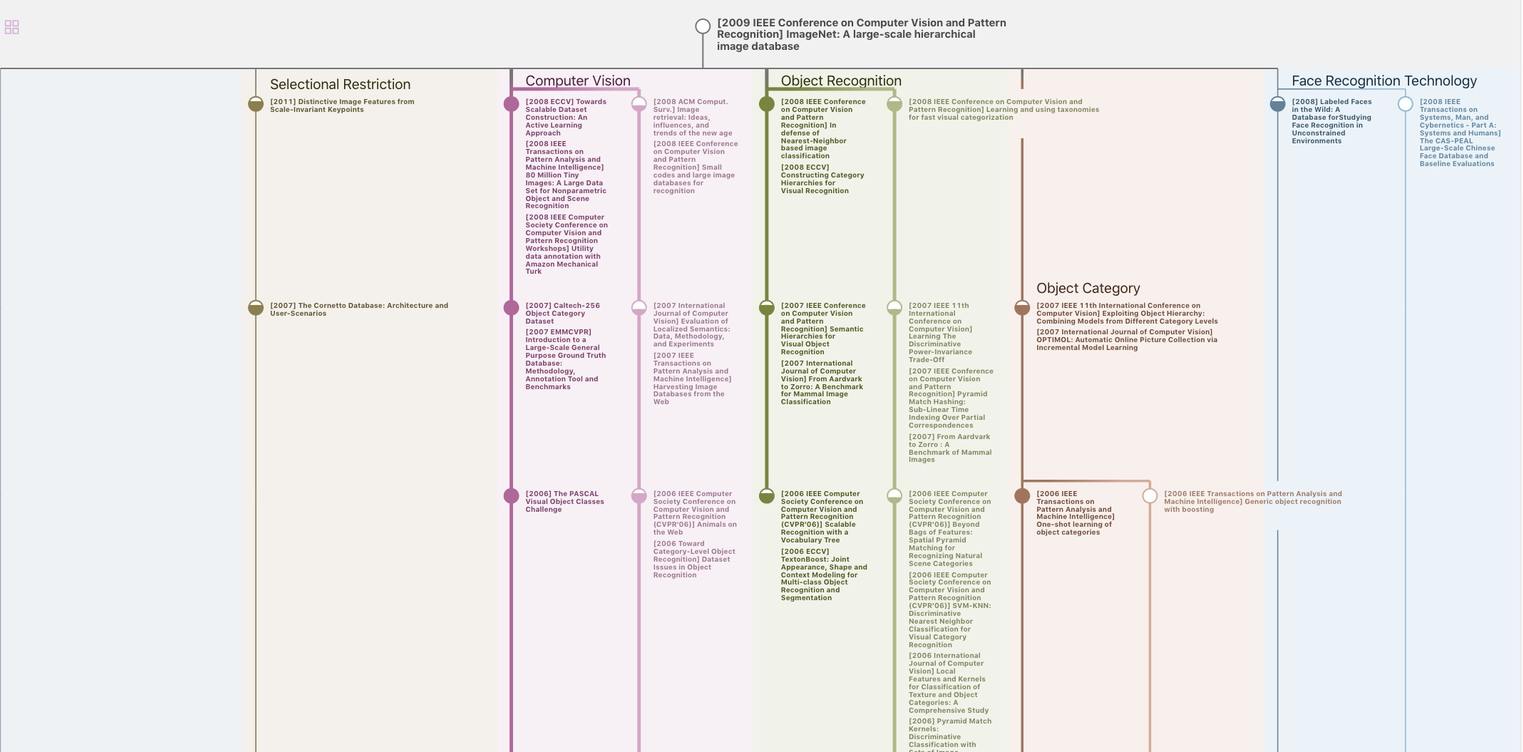
生成溯源树,研究论文发展脉络
Chat Paper
正在生成论文摘要