Contribution of melanin-concentrating hormone (MCH1) receptor to thermoregulation and sleep stabilization: Evidence from MCH1 (−/−) mice
Behavioural Brain Research(2011)
摘要
Recent studies have explored the implication of melanin-concentrating hormone (MCH) in the process of vigilance states. The current experiments were carried out in mice lacking the MCH 1 receptor (−/−) and wild-type (WT) littermates, to assess the role of MCH 1 receptor in the regulation of sleep architecture, body temperature (BT) and locomotor activity (LMA) under normal condition and following a 1 h restraint stress at lights onset. Under baseline conditions, MCH 1 (−/−) mice exhibited consistent changes in waking and sleeping time across the 24-h recording period. We found an increase in the amount of wakefulness (MCH 1 (−/−) 680.1 ± 15.3 min vs. WT, 601.9 ± 18.1, p < 0.05) at the expense of total duration of non rapid eye movement (NREM) sleep (MCH 1 (−/−) 664.1 ± 13.9 min vs. WT 750.1 ± 18.5, p < 0.05). Additionally, MCH 1 (−/−) mice had a higher mean basal body temperature (MCH 1 (−/−), 36.6 ± 0.1° C vs. WT, 36.0 ± 0.1 °C, p < 0.05), particularly during the light-resting period. Restraint stress resulted in an immediate increase in wakefulness with a concomitant reduction in NREM sleep and REM sleep in both genotypes, followed by a homeostatic rebound sleep. A concomitant long lasting increase in BT, independently of the behavioural state accompanied those changes in both genotypes. The elevated basal body temperature and reduction in NREM sleep time resulting from shorter NREM episode durations observed in MCH 1 (−/−) suggests that central MCH 1 receptor has a role in thermoregulation and presumably stabilization of NREM sleep. Keywords Mice Melanin-concentrating hormone MCH1 receptor Sleep Thermoregulation Stress 1 Introduction Melanin-concentrating hormone (MCH), a 19 amino acid peptide neuropeptide is abundantly expressed in neurons of the lateral hypothalamus and zona incerta regions, which have broad fields of projections throughout the central nervous system [1,2] . Rodent pharmacology demonstrated that MCH, by acting through MCH 1 receptors exclusively in the brain [3–5] , plays an important role in the control of feeding behaviour. Acute and chronic central infusion of MCH enhances feeding and hyperphagia [6–8] . Additionally, chronic ablation of the MCH precursor gene in mice resulted in hypophagia, a lean phenotype and increased metabolic rate [9,10] , whereas transgenic animals overexpressing MCH developed mild obesity [11] . In view of its enriched distribution within the brain areas involved in the modulation of mood [1,12] , recent evidence suggested the implication of the MCH system in the control of stress response and anxiety. Central administration of MCH stimulated the activity of the hypothalamic-pituitary adrenal axis (HPA) and produced changes in anxiety related behaviour [13–16] . Acute systemic blockade of the MCH 1 receptor elicited anxiolytic and antidepressants-like effects in behavioural models of anxiety and depression [17–19] . To further support its involvement in mood and anxiety related processes, genetic inactivation of the MCH 1 receptor in mice resulted in an anxiolytic phenotype across a series of commonly used anxiety-related behavioural paradigms [19] . Another higher physiological function attributed to the hypothalamus is the regulation of vigilance states [20] . The MCHergic system overlap in distribution with the orexinergic system, which is associated with a syndrome of narcolepsy [21–24] , however with an opposite functional role. In contrast to stimulating properties of orexin, an increased c-fos activity is present in MCH neurons during sleep recovery indicates the potential role of MCH in dampening arousal pathways [25,26] . In addition, central infusion of MCH had powerful hypnogenic properties [27,28] . Moreover, MCH knockout (MCH−/−) mice slept less during both the light and dark phase of the circadian time [29] . This finding in mice have been supported by a report in rat, in which pharmacological blockade of MCH 1 receptor with two novel MCH 1 receptor antagonists decreased sleep and enhanced wakefulness [30] . In order to further explore the physiological role of the MCH 1 receptor, the present work was undertaken to characterize, under baseline conditions, the sleep–wake architecture, circadian aspects of locomotor activity and body temperature in MCH 1 receptor-deficient mice. Altered sleep timing and sleep architecture are consistently associated with stress, and acute restrain stress at lights-on of the circadian time is widely used in rodents to investigate sleep response to a stressor. A short duration enhancement of arousal at the expense of sleep associated with a delayed rebound compensatory sleep has been described [31–33] . We therefore examined the sleep–wake pattern following 1-h restraint stress applied at the beginning of the light period of the circadian cycle. 2 Materials and methods 2.1 Animal care Founders on 129/SvEV-C57BL/6J background generated MCH 1 null mice, and all animals used in this study had been backcrossed with C57BL/6J for 6–9 generations. In these studies, a total of 16 mice were used for all part of the study: 8 male MCH 1 (−/−) mice and 8 control wild-type (WT) littermates weighing 25–30 g. Mice were maintained under a standard 12:12 light cycle regime (light on 12:00 a.m.; light intensity: ∼100 lx), the temperature was kept at 22 °C ± 2 °C and relative humidity was between 50 and 60%. Standard food chow and tap water were available ad libitum and environmental conditions were controlled). Animals were housed in individually ventilated cages (IVC) located in a sound-attenuated chamber. All experiments were carried out in accordance with the local ethical committee rules for animal experimentation and the European Communities Council Directive of 24 November 1986 (86/609/EEC). 2.2 EEG/BA/LMA recordings and vigilance state analysis Mice were surgically implanted with a transmitter (TA10ETA-F20, Data Science International, USA), containing biopotential electrodes for recording of electroencephalographic, body temperature and locomotor activities (EEG, BT and LMA). All surgical procedures were performed under deep isoflurane anesthesia (4% induction, 1.5% maintenance) and thermostatically controlled body temperature, using a heating pad, throughout the operation and during recovery from anesthesia and surgery. The transmitter was placed in the peritoneal cavity and sutured to the muscle wall with nylon sutures to prevent internal movement. The leads coming from the implant were tunneled from the peritoneal cavity and led subcutaneously to the head in order to fix the end tip of lead to stainless screws for EEG monitoring. The incision in the abdominal wall was sutured with uninterrupted stitches. Afterwards, holes were drilled in the brain skull, AP – 2.3 mm, L ± 1.4 mm from bregma, according to stereotaxic atlas of Paxinos and Franklin [34] and screws were fixed into the skull, covered with dental cement and the skin above the head closed with nylon sutures. After surgery, the animals received subcutaneously 0.3 ml analgesic (Carprofen, Rimadyl, 50 mg/ml, Pfizer Ltd, UK diluted 1:10) and local analgesic on the wounds (Lidocaine spray Xylocaine, 1% solution, Astra Pharmaceuticals Ltd, UK). Subsequently, animals were individually placed in their home cage kept warm in a heating box set at 26 °C ± 2 °C to avoid hypothermia and the temperature was progressively decreased over days until it reached room temperature. The animals were allowed to recover from surgery for at least 2 weeks. In a first study, we have monitored the spontaneous sleep–wake cycle over 24 h after adaptation to the recording conditions. In the second study, we investigated the effects of restraint stress on sleep, BT and LMA. In the restraint stress experiments, the mice were subjected to a 1-h restraint stress in their home cage during the first hour of the light phase. The restraint stress procedure was performed by confining animals in a restraint tube (diameter of 3 cm with holes at both ends for tail and nose). The length of the tube was adjusted to the size of the animals to ensure a complete immobilization. Recordings started by placing the animals in their home cages under the appropriate receivers. The telemetry signals were processed for analogue output by a Data Sciences International analogue converter (Dataquest ART 2.3 Gold version). BT sampled for 10-s was averaged at 5 min intervals and movements in the cage was accounted in 5-min bins. The EEG signals were digitised at a sampling rate of 100 Hz, imported offline into Somnologica 3 software (Stowood, Medcare, Iceland) and digitally filtered (high frequency band 50 Hz and low frequency band at 0.5 Hz) while analysing the vigilance states. The vigilance states were visually scored in 10-s epochs as being either wakefulness, non rapid eye movement (NREM) sleep or rapid eye movement (REM) sleep, based on EEG characteristics and LMA. 2.3 Statistical analysis All data were expressed as mean values ± S.E.M. Statistical significance was evaluated in consecutive hours by using the non-parametric Wilcoxon Mann–Whitney test for 2 independent groups with Bonferroni correction. A value of p < 0.05 was considered to be significant. 3 Results 3.1 Hourly profile of vigilance states, BT and LMA under baseline conditions Both MCH 1 (−/−) and their control WT littermates exhibited a clear-cut circadian sleep–wake rhythm with large amounts of sleep during the light phase as compared to the dark phase of the circadian time. When consecutive hour blocks for the amounts of wakefulness and NREM sleep were compared, a tendency for an increase in wakefulness at the expense of NREM sleep was observed in MCH 1 (−/−) animals ( Fig. 1 a) . Accordingly, the amount of 12-h NREM was significantly decreased in both the light and the dark phases of the circadian time, which parallel the increase in wakefulness observed in the MCH 1 (−/−) animals ( Fig. 1 b). The decrease in NREM sleep time as well as the increase in wakefulness time resulted from shorter NREM episode durations and a trend for longer episodes of wakefulness, while the number of NREM sleep and wakefulness episodes did not reveal consistent changes ( Fig. 1 b). Additionally, the 24-h profile of BT revealed an elevation of basal core body temperature in MCH 1 (−/−) compared to WT mice, which was not uniform throughout the light–dark cycle ( Fig. 2 ). The daily difference in the average of core body temperature between both genotypes showed an increase in basal core BT in MCH1 (−/−) animals (36.6 ± 0.1° C vs. WT, 36.0 ± 0.1 °C, p < 0.05), particularly during the light phase of the circadian time, which seemed to be independent of LMA ( Fig. 2 ). 3.2 Hourly changes in vigilance states, BT and LMA following 1-h restraint stress at the beginning of the light phase Restraint stress applied for 1-h at the beginning of the light phase caused significant changes in different parameters measured in WT littermates ( Fig. 3 a and b) and MCH 1 (−/−) animals (Figs. 4a and b and 5 ). An initial increase in wakefulness in WT and MCH 1 (−/−) mice occurred concomitantly with reductions of NREM sleep and REM sleep ( Figs. 3 and 4 a). Comparing both mouse genotypes for the specific and immediate response to restraint stress revealed differences in magnitude of the effects, which lasted up to 2-h in MCH1 (−/−) animals showing a significantly shorter REM sleep duration in the 1st and 2nd hours of the registration period ( p < 0.05, respectively), shorter NREM sleep duration ( p < 0.05 and p = 0.057, respectively) and longer wakefulness ( p < 0.05 and p = 0.059, respectively). In contrast, the response was confined to the first hour after release from immobilization stress in WT animals. In both genotypes, the initial increase in wakefulness did not modify the total amount of wakefulness during the 12-h light period; however the organisation of this vigilance state was significantly affected during the 12-h light period following stress exposure, as the mean duration with longer episodes of wakefulness while their numbers was reduced ( Figs. 3 and 4 b). The initial suppression of NREM sleep or REM sleep did not change the overall amount of those sleep stages during the entire recording period in both genotypes. However, the suppression of NREM and REM sleep was followed by a rebound hypersomnia during the subsequent dark period, as revealed particularly in WT mice by a significant increase in the 12-h amount of REM sleep ( Fig. 3 b). Under baseline conditions, a significant difference between both genotypes was observed in the mean BT measures during the light phase ( Fig. 5 ), while no significant difference in the average of BT was observed during the 12-h dark period (36.5 °C in WT versus 36.6 °C in MCH 1 (−/−) mice). A similar overall pattern was observed in LMA during the light phase, however no significant difference was found in the average of physical movements between both genotypes ( Fig. 5 ). During the dark phase of the circadian cycle, both genotypes exhibited an approximately two-fold increase in LMA as compared to light phase, with a mild hyperactivity MCH 1 (−/−) mice when compared to WT mice ( Fig. 5 ). After immobilization stress, BT was increased during the light phase in both genotypes to reach maximal comparable temperatures (37.6 °C in WT versus 37.5 °C in MCH 1 (−/−) ( Fig. 5 ). This blunted increase in BT was unlikely associated with changes in LMA, as the mean physical activity values did not diverge significantly ( Fig. 5 ). However, during the dark phase LMA was significantly reduced in response to initial stress exposure ( Fig. 5 ). 4 Discussion 4.1 Baseline conditions In this series of experiments, we have shown that MCH 1 (−/−) mice exhibited a clear-cut circadian sleep–wake rhythm comparable to their WT littermates, with greater amounts of sleep during the light phase than during the dark phase. However, examination of the amounts of NREM and wakefulness across the 12 and 24 h of the light–dark cycle revealed a consistent reduction in NREM sleep time at the expense of wakefulness in MCH 1 (−/−) suggesting that permanent deletion of the MCH 1 receptor could affect the overall sleep–wake profile in both the light and the dark phases. Under baseline conditions, the hourly time course of vigilance states across the 24 h recording period showed a trend for reduction in NREM in favour of wakefulness. Recent reports concerning the potential of MCH to modulate sleep initiation and/or maintenance have suggested a role of this neuropeptide in the regulation of arousal behaviour [25–28] , MCH neurons may function in opposition to other arousal-activating systems in order to dampen arousal and to enhance sleep [25] . In agreement with this hypothesis, c-fos expression revealed a reciprocal pattern of activity between orexinergic and MCH neurons; with MCH cells showed more activation after sleep recovery particularly during non-REM sleep [26] . In keeping with the relationship between MCH neuronal activity and behavioural inactivity or rest, intracerebroventricular infusion of MCH decreased energy metabolism and hypothalamic transmission, which could be associated to biological process observed during sleep [10,35] . An enhancement of NREM sleep and REM sleep was found after central administration of MCH in rats during the dark phase [28] as well as at the beginning of the light phase of the circadian cycle [27] . In addition, indirect evidence showed high activity of MCH neurons during REM sleep [26] . Moreover, MCH knockout (MCH−/−) mice exhibited less sleep during both the light and dark phase of the circadian time [29] . Furthermore, recent pharmacological evidence using two novel MCH 1 receptor antagonists have been shown to decrease sleep in favour of wakefulness in the rat [30] . The sleep–wake cycle has been examined under baseline conditions in a number of gene-manipulated mouse strains, and showed roughly identical circadian patterns and amount of sleep and wakefulness, for instance in the orexin peptides [36] , adenosine A 1 and A 2A receptor [37,38] , and the H 1 receptor [39] mutant mice. Therefore, the rough identical trend in the hourly time course of vigilance states across the 24 h recordings period observed in the present work and findings using various other mouse mutants suggests that sleep and wakefulness processes are highly regulated by different neuronal and humoral pathways to ensure full compensatory mechanisms. In the present work, MCH 1 (−/−) exhibited a global reduction in NREM sleep time in favour of wakefulness when 12-h vigilance states were binned. Our results in MCH 1 (−/−) corroborate with earlier reports in rodents i.e. central infusion of MCH enhance sleep [27,28] consistent with reduction in sleep following MCH1 receptor blockade and deficit in sleep in MCH (−/−) [29,30] . The findings converge into hypnogenic properties of the MCH system by damping the ascending arousal systems possibly through activation of central MCH 1 receptor. In contrast, an increase in REM sleep was reported in MCH 1 (−/−) [40] , which is not in line with earlier reports involving the MCH system in the neurobiological mechanism of sleep. Inconsistent findings have also been reported in behavioural studies associated with an anxiolytic effects observed following increased [41] or decreased in the activity of MCH system [16–19] . MCH 1 (−/−) animals exhibited normal circadian patterns in both BT and LMA, this mutant mouse exhibited higher basal levels of BT during both resting and active phases. A relationship does exist between sleep and thermoregulation. NREM sleep is associated with a consistent fall in temperature, while wakefulness and REM sleep are coupled with increases in brain temperature [42] . In addition, the acrophase of sleep intensity in humans occurs with minimal body temperature associated with peripheral heat loss [43,44] . The fact that MCH 1 (−/−) animals failed to lower their body temperature might explain the global 12-h decreases in NREM sleep characterized by shorter episode of NREM sleep in favour of waking time observed in this mutant. The findings suggested additional support for the role of MCH signalling in the process of thermoregulation. In previous studies, MCH 1 receptor deficient mice have been shown to be, independently of the circadian rhythms, hyperactive during 1-h exploration of an open field both under familiar and unfamiliar conditions [19] . Recent investigations attempted to explain the role of the mesolimbic monoamine system in the mechanisms underlying the enhanced locomotor activity observed in MCH 1 (−/−) mice. Accordingly, genetic inactivation of the MCH 1 receptor may result in supersensitivity and up-regulation of mesolimbic dopamine and norepinephrine transporters [45] . On the other hand, examination of the temporal variation in autonomic activity in MCH 1 (−/−) mice showed a diurnal variation of autonomic mechanisms and energy expenditure independent of increases in locomotor activity, which was limited to the dark phase of the circadian time [46] . Therefore, the increased body temperature appeared to result from the alteration in autonomic regulation of the nervous system as a consequence of increased sympathetic and decreased parasympathetic activity [46] . The results on BT are in line with the later observation as no major alteration was observed in locomotor activity across the light dark cycle, which would trigger an elevation of basal body temperature in MCH 1 (−/−). 4.2 Stress conditions The effects of restraint stress resulted in suppression of NREM sleep and REM sleep during the first 2 h post stress in MCH 1 (−/−) animals, whereas these effects were confined to the first hour post stress in WT littermates and were less pronounced on NREM sleep. A slight compensation of sleep occurred during the light phase following the initial suppression of NREM and REM sleep, while a delayed homeostatic rebound of REM sleep was observed during the subsequent dark phase of the circadian time particularly in WT mice. In rodents, the stress-sleep relationship has long been studied using immobilization stress and sleep deprivation. Acute restraint stress in rats and mice is often accompanied by a short increase in wakefulness and strong suppression of REM sleep followed by consistent delayed increases in REM sleep for several hours attributed to delayed return of corticosterone levels to baseline [31–33] . Recently, a reciprocal relationship between the MCH and orexinergic system has been demonstrated following sleep deprivation with the activity of MCH neurons being increased to dampen arousal and promote sleep. The fact that the MCH neuronal signalling is particularly active during sleep recovery following sleep deprivation [26] may point towards a possible role of the MCHergic system in the homeostasis rebound. In the present work, restraint stress procedure used instead of sleep deprivation did not reveal robust increases in REM sleep beyond baseline levels in MCH 1 (−/−) as compared to WT littermates. In support to our present results on minor consequences of stress in MCH 1 (−/−), permanent deficiency of MCH neuropeptide had similar limited effects on the response to sleep deprivation in the MCH (−/−) animals [29] . Together, the relative small changes in vigilance states observed in MCH 1 (−/−) following stress exposure may indicate that this mouse strain is not responsive to any stressor except food deprivation. In addition, a relationship between sleep rebound after immobilization stress in rat and changes in corticostrone levels has been reported [32,47] . The delayed return of corticosterone levels to baseline after restraint stress exposure could account for minor REM rebound observed in MCH 1 (−/−) animals. Measurement of corticostirone levels after stress exposure in MCH 1 (−/−) is required to verify this hypothesis. Earlier reports in mice showed that pharmacological inactivation of the MCH 1 receptor attenuated the handling stress-induced hyperthermia [16] , and reduced stress-induced anxiety-like behaviour [16,19] . Our findings showed a hyperthermia in this mutant mouse under baseline conditions, and the effects of restraint stress-induced hyperthermia were not attenuated in MCH 1 (−/−) animals. MCH and MCH 1 are located in the hypothalamus, which is a key region of the central nervous system involved in the control of homeostasis, including energy balance and core body temperature. Although the metabolic status was not evaluated in these studies, MCH 1 (−/−) animals might have an increased metabolism rate as it was found in MCH (−/−) [29] , which might explain the hyperthermia that was independent from motor behaviour. Further experiments are indicated to assess metabolic properties in this mutant. In summary, the presented results showed that genetic inactivation of MCH 1 receptor resulted in a marked increase in basal body temperature and reduced NREM sleep time characterized by shorter NREM sleep episodes. Although genotype did not affect number of vigilance states periods under baseline condition, we have noticed genotype differences in sleep architecture at the level of mean duration of NREM sleep episodes that can have a profound impact on sleep stabilization and consolidation. The elevated body temperature under baseline condition or following restraint stress and marginal delayed compensation in sleep suggest that MCH system and MCH 1 receptors have role in thermoregulation and presumably stabilization of NREM sleep. References [1] J.C. Bittencourt F. Presse C. Arias C. Peto J. Vaughan J.L. Nahon The melanin-concentrating hormone system of the rat brain: an immuno- and hybridization histochemical chracterisation J Comp Neurol 319 1992 218 245 [2] G. Skofitsch D.M. Jacobowitz N. Zamir Immunohistochemical localization of a melanin concentrating hormone-like peptide in the rat brain Brain Res Bull 15 1985 635 649 [3] J. Chambers R.S. Ames D. Bergsma A. Muir L.R. Fitzgerald G. Hervieu Melanin-concentrating hormone is the cognate ligand for the orphan G-protein-coupled receptor SLC-1 Nature 400 1999 261 265 [4] P.M.C. Lembo E. Grazzini J. Cao D.A. Hubatsch M. Pelletier C. Hoffert The receptor for the orexigenic peptide melanin-concentrating hormone is a G-protein-coupled receptor Nat Cell Biol 1 1999 267 271 [5] Y. Saito M. Cheng F.M. Leslie O. Cevilli Expression of the melanin-concentrating-hormone receptor mRNA in the rat brain J Comp Neurol 435 2001 26 42 [6] O. Della-Zuana F. Press C. Ortola J. Duhault J.L. Nahon N. Levens Acute and chronic administration of melanin-concentrating hormone enhances food intake and body weight in Wistar and Sprague–Dawley rats Int J Obes Relat Metab Disord 26 2002 1289 1295 [7] D. Qu D.S. Ludwig M. Piper M.A. Pelleymounter M.J. Cullen W.F. Mathes A role for melanin-concentrating hormone in the central regulation of feeding behaviour Nature 21 1996 243 247 [8] T. Suply O. Della Zuana V. Audinot M. Rodriguez P. Beauverger J. Duhault SLC-1 receptor mediates effect of melanin-concentrating hormone on feeding behavior in rat: a structure-activity study J Pharmacol Exp Ther 299 2001 137 146 [9] D.J. Marsh D.T. Weingarth D.E. Novi H.Y. Chen M.E. Trumbauer A.S. Chen Melanin-concentrating hormone 1 receptor-deficient mice are lean, hyperactive, and hyperphagic and have altered metabolism Proc Natl Acad Sci USA 99 2002 3240 3245 [10] M. Shimada N.A. Tritos B.B. Lowell J.S. Flier E. Maratos-Flier Mice lacking melanin-concentrating hormone are hypophagic and lean Nature 396 1998 670 674 [11] D.S. Ludwig N.A. Tritos J.W. Mastaitis R. Kulkarni E. Kokkotou J. Elmquist Melanin-concentrating hormone overexpression in transgenic mice leads to obesity and insulin resistance J Clin Invest 107 2001 379 386 [12] G.J. Hervieu J.E. Cluderay D. Harrison J. Meakin P. Maycox S. Nasir The distribution of the mRNA and protein products of the melanin-concentrating hormone (MCH) receptor gene, slc-1, in the central nervous system of the rat Eur J Neurosci 12 2000 1194 1216 [13] M.I. Gonzalez S. Vaziri C.A. Wilson Behavioral effects of alpha-MSH and MCH after central administration in the female rat Peptides 17 1996 171 177 [14] D. Jezova V. Bartanusz I. Westergren B.B. Johansson J. Rivier W. Vale Rat melanin-concentrating hormone stimulates adrenocorticotropin secretion: evidence for a site of action in brain regions protected by the blood–brain barrier Endocrinology 130 1992 1024 1029 [15] A.R. Kennedy J.F. Todd W.S. Dhillo M.A. Ghatei C.P. O’Toole M. Jones Effect of direct injection of melanin-concentrating hormone into the paraventricular nucleus: further evidence for a stimulatory role in the adrenal axis via SLC-1 Neuroendocrinology 15 2003 268 272 [16] D.G. Smith R.J. Davis L. Rorick-Kehn M. Morin J.M. Witkin D.L. McKinzie Melanin-concentrating hormone-1 receptor modulates neuroendocrine, behavioral, and corticolimbic neurochemical stress responses in mice Neuropsychopharmacology 31 2006 1135 1145 [17] B. Borowsky M.M. Durkin K. Ogozalek M.R. Marzabadi J. DeLeon B. Lagu Antidepressant, anxiolytic and anorectic effects of a melanin-concentrating hormone-1 receptor antagonist Nat Med 8 2002 825 830 [18] S. Chaki T. Funakoshi S. Hirota-Okuno M. Nishiguchi T. Shimazaki M. Iijima Anxiolytic- and antidepressant-like profile of ATC0065 and ATC0175: nonpeptidic and orally active melanin-concentrating hormone receptor 1 antagonists J Pharmacol Exp Ther 313 2005 831 839 [19] M. Roy N.K. David J.V. Danao H. Baribault H. Tian M. Giorgetti Genetic inactivation of melanin-concentrating hormone receptor subtype 1 (MCHR1) in mice exerts anxiolytic-like behavioral effects Neuropsychopharmacology 31 2006 112 120 [20] C.B. Saper T.E. Scammell J. Lu Hypothalamic regulation of sleep and circadian rhythms Nature 27 2005 1257 1263 [21] R. Chemelli J. Willie C. Sinton J. Elmquist T. Scammell C. Lee Narcolepsy in orexin knockout mice. Molecular genetics of sleep regulation Cell 4 1998 437 451 [22] L. Lin J. Faraco R. Li H. Kadotani W. Rogers X. Lin The sleep disorder canine narcolepsy is caused by a mutation in the hypocretin (orexin) receptor 2 gene Cell 3 1998 365 376 [23] C. Peyron D.K. Tighe A.N. van den Pol L. de Lecea H.C. Heller J.G. Sutcliffe Neurons containing hypocretin (orexin) project to multiple neuronal systems J Neurosci 18 1998 9996 10015 [24] C. Peyron J. Faraco W. Rogers B. Ripley S. Overeem Y. Charnay A mutation in a case of early onset narcolepsy and a generalized absence of hypocretin peptides in human narcoleptic brains Nat Med 6 2000 991 997 [25] L. Bayer E. Eggermann M. Serain J. Grivel D. Machhard M. Muhlethaler Opposite effects of noradrenaline and acetylcholine upon hypocretin/orexin versus melanin concentrating hormone neurons in rat hypothalamic slices Neuroscience 130 2005 807 811 [26] M. Modirrousta L. Mainville B.E. Jones Orexin and MCH neurons express c-Fos differently after sleep deprivation vs. recovery and bear different adrenergic receptors Eur J Neurosci 21 2005 2807 2816 [27] A. Ahnaou W.H.I.M. Drinkenburg H. Huysmans A. Heylen T. Steckler F.M. Dautzenberg Differential roles of hypothalamic neuropeptides in sleep–wake modulation in rats Soc Neurosci Meeting Abstr 2006 P458.1 [28] L. Verret R. Goutagny P. Fort L. Cagnon D. Salvert L. Léger A role of melanin-concentrating hormone producing neurons in the central regulation of paradoxical sleep BMC Neurosci 4 2003 19 28 [29] J.T. Willie C.M. Sinton E. Maratos-Flier M. Yanagisawa Abnormal response of melanin-concentrating hormone deficient mice to fasting: hyperactivity and rapid eye movement sleep suppression Neuroscience 156 2008 819 829 [30] A. Ahnaou F.M. Dautzenberg H. Geys H. Imogai A. Gibelin D. Moechars Blocking melanin-concentrating hormone MCH1 receptor affects rat sleep–wake architecture Eur J Pharmacol 579 2008 177 188 [31] C. Bonnet L. Leger V. Baubet G. Debilly R. Cespuglio Influence of a 1-h immobilization stress on sleep states and corticotrophin-like intermediate lobe peptide (CLIP or ACTH18-39, Ph-ACTH18-39) brain contents in the rat Brain Res 75 1997 54 63 [32] S. Marinesco C. Bonnet R. Cespuglio Influence of stress duration on the sleep rebound induced by immobilization in the rat: a possible role for corticosterone Neuroscience 92 1999 921 933 [33] G. Vazquez-Palacios J. Velazquez-Moctezuma Effect of electric foot shocks, immobilization, and corticosterone administration on the sleep–wake pattern in the rat Physiol Behav 7 2000 23 28 [34] G. Paxinos K.B.J. Franklin The mouse brain in stereotaxic coordinates 2nd ed. 1997 Academic Press San Diego, California [35] A.N. Van den Pol C. Acuna-Goycolea K.R. Clark P.K. Ghosh Physiological properties of hypothalamic MCH, neurons identified with selective expression of reporter gene after recombinant virus infection Neuron 42 2004 635 652 [36] T. Mochizuki A. Crocker S. McCormack M. Yanagisawa T. Sakurai T.E. Scammell Behavioral state instability in orexin knock-out mice J Neurosci 24 2004 6291 6300 [37] Z.L. Huang W.M. Qu N. Eguchi J.F. Chen M.A. Schwarzschild B.B. Fredholm Adenosine A2A, but not A1, receptors mediate the arousal effect of caffeine Nat Neurosci 8 2005 858 859 [38] D. Stenberg E. Litonius L. Halldner B. Johansson B.B. Fredholm T. Porkka-Heiskanen Sleep and its homeostatic regulation in mice lacking the adenosine A1 receptor J Sleep Res 12 2003 283 290 [39] Z.L. Huang T. Mochizuki W.M. Qu Z.Y. Hong T. Watanabe Y. Urade Altered sleep–wake characteristics and lack of arousal response to H3 receptor antagonist in histamine H1 receptor knockout mice Proc Natl Acad Sci USA 21 2006 4687 4692 [40] A. Adamantidis D. Salvert R. Goutagny B. Lakaye D. Gervasoni T. Grisar Sleep architecture of the melanin-concentrating hormone receptor 1-knockout mice Eur J Neurosci 27 2008 1793 1800 [41] J. Kela P. Salmi R. Rimondini-Giorgini M. Heilig C. Wahlestedt Behavioural analysis of melanin-concentrating hormone in rats: evidence for orexigenic and anxiolytic properties Regul Pept 114 2003 109 114 [42] S.F. Glotzbach H.C. Heller Temperature regulation M.H. Kryger T. Roth W.C. Dement Principles and practice of sleep medicine 2000 Saunders Philadelphia 289 304 [43] D.J. Dijk C.A. Czeisler Contribution of the circadian pacemaker and the sleep homeostatic to sleep propensity, sleep structure, electroencephalographic slow waves, and sleep spindle activity in humans J Neurosci 15 1995 3526 3538 [44] K. Kräuchi C. Cajochen E. Werth A. Wirz-Justice Functional link between distal vasodilation and sleep-onset latency? Am J Physiol Regul Integr Comp Physiol 278 2000 R741 R748 [45] D.G. Smith E.T. Tzavara J. Shaw S. Luecke M. Wade R. Davis Mesolimbic dopamine super-sensitivity in melanin-concentrating hormone-1 receptor-deficient mice J Neurosci 26 2005 914 922 [46] A. Åstrand Y.M. Bohlooly S. Larsdotter M. Mahlapu H. Andersén J. Tornell Mice lacking melanin-concentrating hormone receptor 1 demonstrate increased heart rate associated with altered autonomic activity Am J Physiol Regul Integr Comp Physiol 287 2004 R749 R758 [47] G.J. Kant R.H. Pastel R.A. Bauman G.R. Meininger K.R. Maughan T.N. Robinson Effects of chronic stress on sleep in rats Physiol Behav 57 1995 359 365
更多查看译文
关键词
Mice,Melanin-concentrating hormone MCH1 receptor,Sleep,Thermoregulation,Stress
AI 理解论文
溯源树
样例
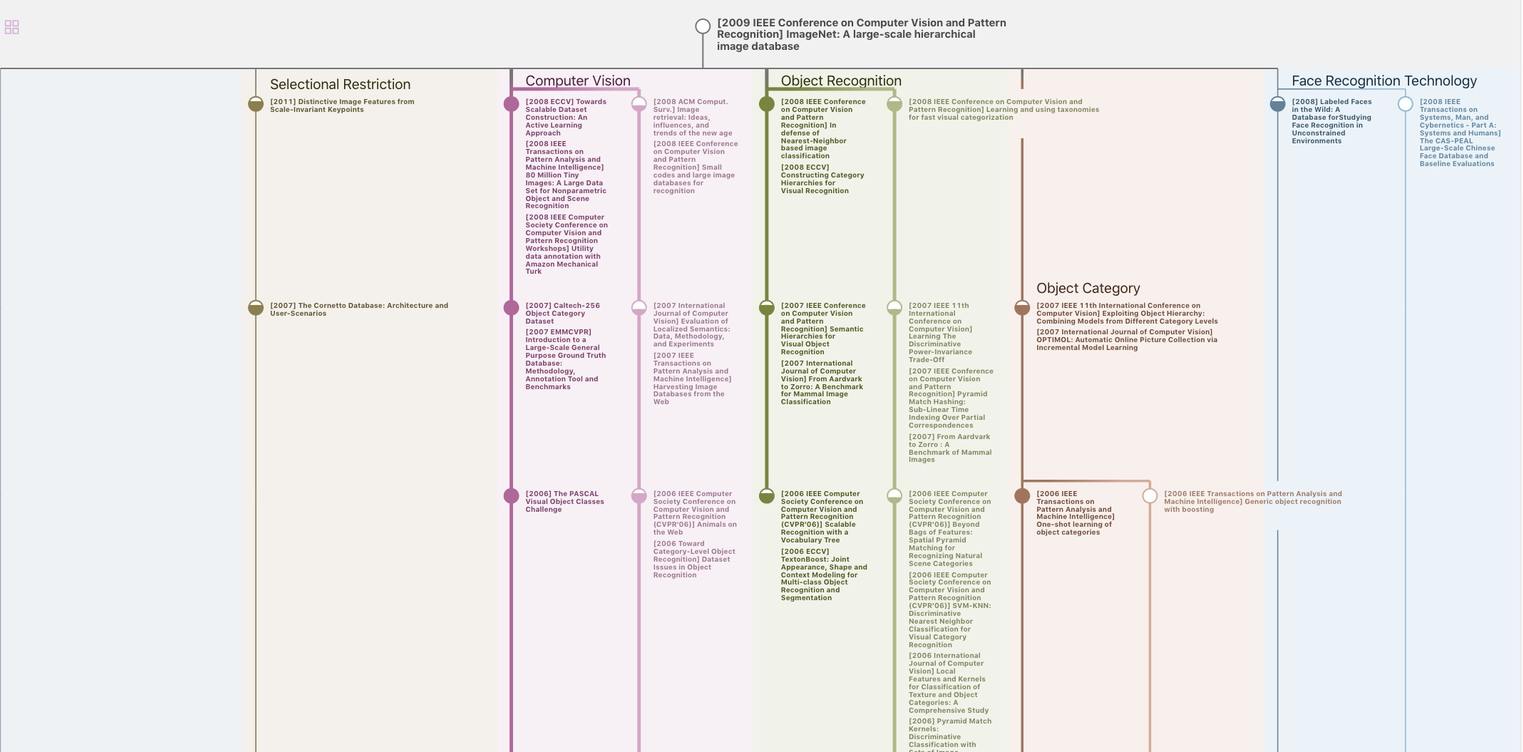
生成溯源树,研究论文发展脉络
Chat Paper
正在生成论文摘要