Single-subject voxel-based relaxometry for clinical assessment of temporal lobe epilepsy
Epilepsy Research(2009)
摘要
Methods Forty-five patients with TLE confirmed by history, EEG, and structural MRI and 25 control subjects were scanned at 3 T using a modified Carr–Purcell–Meiboom–Gill MR sequence. ROIs were drawn for each patient and control subject, and measurements were made on unregistered T2 maps. VBR was performed on a single-subject basis at a significance level of α = 0.05. Patients were grouped according to seizure focus (left mesial, right mesial, other), and whether structural MR imaging was normal or abnormal. Results Up to 85% of patients in the temporal lobe groups demonstrated T2 abnormalities. VBR detected abnormalities either in equal numbers or in more patients (up to 23% more) than ROI analysis for each group. The number of detected abnormalities per patient was higher using VBR (3.38 versus 2.04, p < 0.05). VBR also identified abnormalities that were missed by ROI analysis. The rate of VBR detection of abnormalities was higher for patients than controls (76% versus 36%). Conclusions VBR can be performed on single subjects with TLE and it detects considerably more abnormalities than ROI analysis. VBR may be a clinically useful tool for the detection of T2 abnormalities at the seizure focus and sites remote from it. Keywords Hippocampal sclerosis Partial seizures Temporal lobe epilepsy Epilepsy MRI Relaxometry Introduction Temporal lobe epilepsy (TLE) is the most common form of refractory epilepsy in adults, and hippocampal sclerosis (HS) is a common cause of TLE ( Stafstrom, 2006; Van Paesschen et al., 1997 ). HS can be detected with magnetic resonance (MR) imaging by hippocampal T2 hyperintensity, volume reduction, and loss of gray matter/white matter differentiation ( Jackson et al., 1990, 1993a ). Pathologically, HS is characterized by neuronal loss and gliosis, particularly in areas CA1, CA3 and CA4 in the hippocampus ( Cascino et al., 1991; Meiners et al., 1994 ). Increased T2 relaxation seen on MR corresponds to these pathologic abnormalities ( Grunewald et al., 1994; Jackson et al., 1993b ). T2 is an intrinsic MR property of tissue and quantitative T2 relaxometry (assessing measurement of T2 relaxation time) is a sensitive tool for identifying T2 abnormalities in TLE ( Bernasconi et al., 2000; Mueller et al., 2007; Jackson et al., 1993b ). This technique is more sensitive than visual assessment of MR images alone ( Bernasconi et al., 2000 ). HS presents a clear case where T2 changes may be identified, but abnormalities beyond the hippocampus, some of which may subtle or absent in structural MR, may also be identified by T2 relaxometry. In patients with HS, T2 abnormalities have been detected not only in the hippocampus, but in the adjacent temporal lobe white matter and in remote locations such as the thalamus and in the basal ganglia ( Briellmann et al., 2004a,b; Townsend et al., 2004; Mueller et al., 2007 ). These abnormality findings correlate well with functional and metabolic studies ( Mueller et al., 2004, 2007 ). Overall, these results suggest that seizures have remote structural effects on the brain, and that T2 relaxometry can identify HS and other abnormalities. T2 maps are commonly generated from a multi-echo acquisition, such as a Carr–Purcell–Meiboom–Gill (CPMG) sequence ( Pell et al., 2006a ) or a dual spin echo (DSE) sequence ( Duncan et al., 1996; Jack, 1996 ). The T2 value at each voxel location can be extracted from an exponential curve that is fit to describe the T2-weighted signal decay of the multiple signals acquired at each location. The standard procedure for T2 measurement (T2 relaxometry) uses manually drawn regions of interest (ROIs). The ROI approach is limited by the subjective nature of selecting ROIs and is also restricted in its scope of investigation to selected regions of the brain. Recently, a voxel-based analysis approach termed voxel-based relaxometry (VBR) has been developed, which provides an unbiased statistical analysis of T2 values over the whole brain ( Pell et al., 2004 ). VBR can depict the shape, location, and extent of a T2 abnormality. VBR has been used to study groups of patients with TLE and definite HS based on conventional review of MR scans and on ROI-based T2 relaxometry ( Mueller et al., 2007; Pell et al., 2004 ). These studies demonstrated widespread ipsilateral temporal and some extratemporal abnormalities. The efficacy of single-subject VBR has been demonstrated in one published study at 1.5 T in patients with different seizure etiologies ( Rugg-Gunn et al., 2005 ). Increased T2 signal was seen at sites with obvious structural abnormalities (e.g., acquired lesion or malformations of cortical development) and at the presumed seizure focus in most patients with normal MR scans. It is important to note that most VBR studies have been restricted to selective patient cohorts. Furthermore, studies using VBR are technically limited by partial brain coverage, or T2 acquisition strategies that have compromised accuracy to stay within a desired scan time. None of these limitations disqualify the application of VBR, but may restrict the clinical utility of single subject. Our objective was to assess the clinical utility of whole brain, single-subject VBR at 3 T for a consecutive series of patients with TLE whose structural MRI may or not be normal. We also compared the efficacy of VBR to ROI analysis on single patients. Methods Patients and MR imaging We studied 45 consecutive patients with TLE confirmed by history, EEG, conventional structural MR, and neuropsychological testing (26 female, mean age of 40 ± 13 years, range 22–66 years). Patients provided written informed consent. Research imaging was performed in addition to a routine MR seizure protocol at 3 T (Signa VH/i; General Electric Health Care, Waukesha, WI). Based on clinical data, patients were categorized as follows: right mesial TLE, left mesial TLE, and other (right or left non-mesial TLE). All patients were then categorized into abnormal and normal structural MR groups. T2 relaxometry using a CPMG sequence was performed on all patients. Our CPMG sequence acquires images at eight echo times (TE) for each slice (TE times ranged from 30 ms to 240 ms with equal intervals of 30 ms) with a repetition time TR = 2175 ms, a 256 × 128 acquisition matrix (interpolated to 256 × 256), a 24 cm field-of-view, 15–24 slices of 5-mm thickness separated by a 1-mm gap, and acquired in an oblique coronal plane perpendicular to the long axis of the hippocampus. The CPMG sequence was also performed on 25 healthy control subjects (14 female, mean age of 26 ± 5.4 years, range 18–42 years) who also provided written informed consent. All human imaging followed a protocol approved by our local research ethics board. Processing and analysis ROI analysis T2 maps were generated by extracting fitted T2 parameters from the eight-echo decay curves at each voxel using a Levenberg–Marquardt non-linear fitting routine. The decay curve was defined as S (TE) = S (0) exp(−TE/T2) + k , where S (TE) is the signal acquired at each echo time, TE, and k is a baseline constant that may be included to account for partial volume averaging between brain tissue and cerebrospinal fluid (CSF) ( Pell et al., 2004 ). Two sets of T2 maps were generated: (1) T2 maps with no baseline correction for ROI analysis and CSF segmentation and (2) T2 maps with an optimized voxel-by-voxel baseline fit where the baseline was included if it reduced the residual error present in the non-linear fit estimate. ROIs were placed bilaterally in temporal and extratemporal areas at specific sites that have been studied previously ( Briellmann et al., 2004a; Pell et al., 2004 ). ROI placement is depicted in Fig. 1 . These regions were placed on T2-weighted images (2nd echo, TE = 60 ms) and then copied to the T2 maps for measurement of the mean T2 relaxation values in these regions. Paired student's t -tests were performed to compare hemispheric differences in controls ( α = 0.05). A T2 abnormality in patients was defined as a measurement greater than 1.65 standard deviations. To serve as a reference for false-positive ROI findings, the same ROI abnormality detection scheme was performed on each individual control subject against the remainders of the controls. To determine the possible effect of age, a linear regression analysis was performed for age versus T2 value for each ROI in the control group and across all patients ( α = 0.05). VBR analysis Fig. 2 summarizes the VBR analysis procedure. First, the T2 maps were spatially normalized to a common stereotactic space via non-linear coregistration of T2-weighted images (3rd echo, TE = 90 ms) to a T2 template (provided with SPM2, Wellcome Department of Imaging Neuroscience, www.fil.ion.ucl.ac.uk/spm , 2004). The spatially normalized T2 maps were subsequently smoothed using a Gaussian filter (6 mm, full-width half-maximum). A CSF mask image was produced for each patient dataset by thresholding out regions with T2 > 160 ms on the first set of T2 maps in order to identify CSF regions for subsequent removal ( Fig. 2 ). A brain mask was produced by using the brain extraction tool in FSL (FMRIB Software Library, www.fmrib.ox.ac.uk/fsl , 2004) on the normalized 3rd echo TE = 90 ms image. A final, combined mask was produced from the union of the CSF and brain masks in order to define the voxels in the brain (excluding CSF structures) to be subsequently analyzed. Our selected smoothing and registration parameters have previously been shown to be appropriate for VBR ( Pell et al., 2006b ). Statistical analysis of the smoothed T2 maps was performed using a two-sample student's t -test between the control patient group and individual patients using SPM2 ( α = 0.05). To serve as a reference for false-positive VBR findings, the same statistical analysis was performed for each control versus the remainder of the controls. To determine the possible effect of age on T2, voxel-based statistical analysis was also performed using a linear regression with age as the covariate in the control group and across all patients ( α = 0.05). A VBR severity score was determined based on the presence of VBR significance in any of the studied ROI regions, in addition to the following extra regions for each hemisphere: anterior temporal lobe grey matter, posterior temporal lobe, frontal lobe grey matter, parietal lobe, occipital lobe, internal capsule and insular ribbon. Thus, eight ROIs and seven additional regions per hemisphere were considered for VBR scoring, giving a maximum score of 30 for both hemispheres. Comparison of ROI and VBR analyses We identified ROIs that had abnormal T2 values and looked at the same region in the VBR analysis. If an ROI had an abnormal T2 measurement that was not apparent on VBR, then this was judged to be an “undercall” by VBR (i.e., VBR failed to identify an area of abnormal T2 relaxation that was identified by ROI analysis). If, however, VBR identified an area of abnormality (either within any of the ROIs or outside the ROIs) that was not seen by ROI analysis, this was judged to be an “overcall” by VBR. We defined extra regions from the presence of any significance in the seven areas not considered for ROI analysis. Non-parametric Wilcoxon signed-rank tests were performed to determine any significant differences between the number of abnormalities detected per patient with ROI analysis versus VBR analysis ( α = 0.05). Results The quantitative, patient-specific ROI measurements for control subjects are summarized in Table 1 . A significant difference between left and right patient-specific T2 values was seen in the frontal lobe white matter, caudate and putamen ( p < 0.05). Because of these differences, control ROI measurements from the left and right hemispheres were not pooled. These differences may have been produced by a bias field effect where the magnetic field (and therefore MR signal) was not uniform throughout the entire image. Note, however, that this variation was systematic, and remained relatively constant between control subjects. Fig. 1 shows box and whisker plots of temporal lobe ROI locations and shows that the values are normally distributed. Table 2 summarizes the comparison of ROI and VBR approaches. For controls, ROI analysis showed 10 of the 25 controls (40%) having at least one abnormality in the temporal lobes, with 13 of the 25 controls (52%) showing an abnormality in any of the studied regions. VBR showed a similar detection rate with 9 (36%) being detected with a temporal lobe abnormality and 11 (44%) having an abnormality in any of the studied regions. The average number of regions showing abnormalities was slightly higher for VBR analysis than for ROI analysis (1.60 abnormalities/control versus 1.24 abnormalities/control, Table 2 ). Only 0.36 extra regions per control were detected, i.e., on average only about one additional abnormality was detected by VBR beyond the ROI analysis for every three patients. Relative to controls, both ROI and VBR analysis exhibited a much higher rate of abnormality detection. ROI analysis showed a modest correlation between the presence of T2 abnormalities in the temporal lobe and the location of the presumed seizure focus. Specifically, 8 out of 13 patients (62%) in the left mesial temporal group showed elevated T2 in the temporal lobe ROIs (6 ipsilateral, 2 contralateral) while the right mesial temporal group yielded similar findings. For the temporal other group, 9 of 16 (56%) patients exhibited elevated T2 in either temporal lobe. A similar correlation with the presumed seizure focus was seen for VBR for the left mesial temporal patients, where 10 patients (77%) showed temporal lobe abnormalities (7 ipsilateral, 3 contralateral) with similar findings for the right mesial temporal group. Twelve of the temporal other group (75%) showed elevated VBR in the temporal lobes. Table 2 shows the overall occurrence of abnormalities in the temporal and extratemporal regions across all three temporal lobe groupings. Abnormalities were observed in equal frequency for the right mesial temporal group and temporal other group (both 75%), but at a higher frequency with VBR in the left temporal group (85% versus 62%). The average number of regions showing abnormalities for the three groups was consistently higher using VBR and when all patients were grouped together this number significantly higher using VBR (3.38 versus 2.04). There was a trend to significance for the temporal lobe groupings. Compared to ROI analysis, VBR identifies more regions of abnormal T2 values than it misses. For the total grouping, 2.29 overcalls per patient versus 0.96 undercalls per patient were observed. The average number of extra regions identified by VBR ranged between 1.06 and 1.23 per patient ( Table 2 ). Fig. 3 shows elevated T2 relaxation times in temporal lobe structures in a patient with right mesial TLE and HS with ROI abnormalities in both hippocampi and the right anterior temporal lobe white matter. VBR also showed these abnormalities but also detected others including right amygdala and remote sites such as right middle and inferior temporal gyri, and left amygdala and temporal lobe white matter ( Fig. 3 ). In another patient, a region of suspected encephalomalacia detected in the structural MR images was clearly revealed using VBR, with T2 abnormalities extending from the right hippocampus to the overlying temporal neocortex ( Fig. 4 ). These cases illustrate the advantage of VBR depicting the shape, location, and extent of an abnormality. A comparison of ROI and VBR measures for patients with normal or abnormal MR scans is shown in Table 2 . T2 abnormalities were seen less often in the normal MR group compared to the abnormal MR group in the temporal lobes (54% versus 84% for VBR and 46% versus 69% for ROI; Table 2 ) with similar findings for the temporal and extratemporal regions combined. There were more overcalls per patient in the normal MR group (4.15 versus 2.47). VBR also detected abnormalities more often than using ROI analysis in the patients with abnormal MR (84% versus 75% for the temporal and extratemporal regions combined). A difference between the detection rate between VBR and ROI analysis occurred with normal MR in the temporal lobes, but there was an equal detection rate for the temporal and extratemporal regions combined (62% for both). The number of abnormalities seen per patient was significantly higher for VBR analysis than for ROI analysis for the abnormal MR group, but this difference was not significant for the normal MR group. There were more overcalls per patient in the normal MR group (4.15 versus 2.47) and the normal MR group revealed more extra regions per patient (1.38 versus 1.00). We found that the controls and patients were significantly different in terms of age ( p < 0.001, Student's t -test). ROI analysis in control subjects showed a significant age effect in the right putamen ( p < 0.05), however, R 2 = 0.2601, indicating only a modest correlation between age and T2. All other R 2 values for controls were less than 0.2, indicating weak correlations between age and the respective T2 values. SPM analysis revealed some significance in both putamina. ROI analysis in the patient group showed significance in the left and right thalami as well as the left putamen and hippocampus. All R 2 values in these areas, however, were less than 0.2, suggesting weak correlations between age and T2 values. SPM analysis also showed some significance in the left hippocampus as well as in broad areas in tissue directly adjacent to CSF. Discussion We compared two different approaches (ROI and VBR) to detect T2 relaxation abnormalities in patients with temporal lobe epilepsy. Notably, this comparison was carried out on a single-subject basis, as we were interested in comparing the clinical utility of the two techniques. We focused on the sensitivity of the techniques—how often each technique detects an abnormality. While ROI analysis inherently limits the scope of investigation, VBR allows for objective whole brain analysis. In some of our patients, ROIs missed regions that showed elevated T2 values based on VBR analysis. For instance, elevated T2 values were identified in parts of the hippocampus posterior to the ROIs for some patients (data not shown). Fig. 3 shows how broad, confluent areas of T2 abnormalities can be identified using VBR, whereas this would not be appreciated with ROIs. Table 2 summarizes the comparison of ROI and VBR approaches. The average number of regions showing abnormalities was a little higher for ROI analysis, resulting in somewhat higher overcalls than undercalls. The control results provide a reference for the rate of false-positive detection for the ROI and VBR methods, as any detected abnormality in the control data can be seen as a false-positive result. It should be noted that one control subject exhibited widespread T2 abnormalities (abnormal T2 with VBR in all studied ROI areas), which heavily influenced the tabulation of abnormalities per patient. Such occurrences of extensive T2 differences may occur, and be valid deviations within a normal non-epileptic population. Thus, it is important to not discard such data out of hand, especially considering how the reliability of T2 mapping has been shown to be high ( Peran et al., 2007 ). We compared VBR and ROI analysis in TLE patients. We found that more patients showed a temporal lobe abnormality with VBR analysis ( Table 2 ). Thus, VBR is more likely to detect temporal lobe abnormalities than ROI analysis. VBR also identified more abnormalities per patient. Furthermore, for all patients, 1.11 extra T2 abnormalities per patient were seen using VBR compared to ROI analysis ( Table 2 ). These results demonstrate the advantage of whole brain analysis since abnormalities not picked up by ROI analysis can be detected with VBR. It is also important to note that compared to ROI analysis, the VBR control data had a lower detection rate. This makes the sensitivity of VBR detection in patients higher than for ROI analysis if the control data is used as a reference for the incidence of false-positive detections. Our data supports the clinical use of VBR, however further confirmation of its utility should be evaluated through a study of post-operative outcome in patients who had and did not have resections of areas deemed to be abnormal by VBR. Our control values ( Table 1 ) are systematically lower than those reported previously ( Pell et al., 2004; Briellmann et al., 2004a ), likely attributable to small differences in scanner hardware and the CPMG sequence implementation. This highlights the importance of having control and patient data acquired using the same scanner and pulse sequences if T2 relaxometry is to be used clinically. We observed differences between left and right ROI measurements in control subjects that can be attributed to a bias field effect. T2 values should ideally be independent of such a systematic effect, however noise effects at later time points of the T2 relaxation curve may reveal the bias field effect. Such differences should not adversely affect the validity of VBR analysis when using the same scanner, as the bias field effect should be consistent across patients and controls with the relative difference in T2 values between the two groups determining the level of significance. One major challenge of single-subject VBR is the influence of random artifacts or patient-specific features. An example of this limitation is CSF partial volume averaging along the gyri and sulci (see Fig. 4 ). Also, there is the requirement to mitigate even small, localized partial volume averaging effects, such as those found around the hippocampus through an approach such as a baseline correction. Therefore, although VBR is inherently objective, it is necessary to be aware of possible sources of error. In a group analysis, these non-uniform artifacts are cancelled out by averaging across patients. Despite this advantage, group VBR analysis has a number of disadvantages. Meaningful group analysis can only be performed on homogeneous patient groups, which limits the number or types of patients that can be studied. In addition, the averaging process can nullify sporadic abnormalities that may be seen only in a subset of patients. This disadvantageous cancellation is analogous to advantageous cancellation of the cortical CSF artifact described above. Another important consideration with VBR (as with any voxel-based statistical test) is the multiple comparisons problem where false-positive detections occur ( Kiebel et al., 1999; Worsley, 2003 ). The inherently limited resolution of the acquisition, reslicing from registration, and Gaussian smoothing produces voxel-level intensity values that are non-independent. Note that with independent T2 values – no smoothing or averaging effects – our single-subject analysis should simply yield a false-positive detection rate equal to the level of significance ( α = 0.05). Considering the signal-averaging effects, false-positive detections can be limited to a specified proportion of detections using false discovery rate thresholding (usually limited to 5%), but this technique tends to be conservative ( Worsley, 2003 ). Alternatively, using Gaussian random field theory, a family-wise error correction can be applied to accept a certain probability of a false-positive detection occurring based on the number of voxels and underlying smoothness of the statistical parametric map. This correction, however, requires the spatial distribution of statistical significance to be Gaussian, which requires a sufficient amount of smoothing to ensure this assumption is upheld—possibly with detriment of sensitivity to small abnormalities. In this analysis, we did not perform either correction since they proved to be far too conservative for our data, and virtually limited all significant findings in most patients. Though our data underwent moderate smoothing (in our case 6 mm Gaussian kernel, full-width half-maximum), high image contrast and a lack of signal averaging (which would normally occur with a group comparison) rendered these techniques unsuitable. One must be mindful of potentially introducing a substantial false-negative error while trying to correct for false-positives. In our case, we opted for the presence of false-positive detections over introducing a large (and uncharacterized) false-negative error, so we set the threshold relatively high ( α = 0.05, one-tailed). As a result, the VBR detection of abnormalities in the temporal lobe regions for controls was somewhat high at 36%. A correction for multiple comparisons may reduce this detection rate, but it is important to note that our detections rates are based on the tabulation of individual region findings. This may be deemed acceptable because our primary goal is to determine where any abnormalities exist, as well as if any abnormalities exist. This would be consistent with the idea that VBR is most useful for characterizing abnormalities spatially, and not for a confirmation of an epilepsy diagnosis itself. Age presents a potential confounding factor. For controls, we found a significant age effect in the putamina, which may have impacted our ROI and VBR T2 findings depending on the patients’ ages. SPM analysis for the patients revealed broad significance in tissue adjacent to CSF, which is consistent with age-related cerebral atrophy in some of the older subjects in our patient group. As CSF cannot be perfectly excluded, and as registration can only partially reduce inter-subject partial volume effects between CSF and GM, this result is to be expected. For the patient group, factors such as the duration of epilepsy (which is directly related to age) and seizure frequency may confound this analysis, and brings into question the validity of the finding of a significant age effect in the left hippocampus for patient group. A study with healthy controls spanning a larger age range is necessary for a robust determination of the effect of age on T2. Single-subject VBR relies heavily on accurate registration and T2 estimates since inaccurate T2 estimations may not be recognized by visual inspection. The CPMG sequence is more accurate than the DSE sequence, however, the practical significance of this advantage has been debated ( Duncan et al., 1997; Jack, 1996 ). One issue with the CPMG sequence is inaccuracy in the 180-degree pulses, which can lead to compounded error and stimulated echoes. We noticed the possible manifestation of stimulated echoes in some of our data as a mild band of artifact passing parasagittally through the central region of the brain. However, the CPMG sequence allows for more points to be fitted (in our case 8); DSE uses only 2 points for fitting, so there may be a greater potential for error ( Jack, 1996 ). We believe that accuracy is critical for VBR. Therefore any potential gains in accuracy by using the CPMG sequence should be considered important for clinical purposes. Patient-specific ROIs should be drawn in patients with normal conventional MR scans or in patients where accurate registration is not possible due to patient-specific anatomical variations or image artifact. An optimal ROI analysis protocol would require a broad scope of ROIs to ensure adequate coverage, with a priori knowledge of appropriate locations. However, drawing a large number of ROIs for every individual may be unfeasible in a clinical setting. For a given structure, multiple ROIs can drawn to increase local coverage as has been done previously ( Townsend et al., 2004; Bernasconi et al., 2000 ). However, this may not ultimately increase the sensitivity of the method as abnormalities in one ROI may be cancelled by averaging with other ROIs drawn in normal parts of the same structure. Although VBR is more technically challenging and much more intensive to establish, once it is in routine use, VBR analysis requires less operator interaction and decision-making than an extensive ROI analysis. Furthermore, it is possible for VBR maps to be generated in a semi-automated fashion. Ultimately, we believe that VBR analysis of T2 maps may be more clinically effective than ROI analysis owing to its objectivity, whole brain assessment, and minimal user interaction. VBR can also depict the extent of abnormality and can reveal remote abnormalities better than ROI analysis, particularly in patients with highly abnormal T2 values. Acknowledgements This work was supported by the Alberta Heritage Foundation for Medical Research (AHFMR), Canada Foundation for Innovation (CFI), Canadian Institutes of Health Research (CIHR). R.K.K. is supported by AHFMR, Natural Sciences and Engineering Research Council of Canada, and Informatics Circle of Research Excellence Graduate Scholarship awards. R.F. is a Canada Research Chair and an AHFMR Senior Scholar. P.F. is a CIHR Clinician-Scientist and an AHFMR Clinical Investigator. References Bernasconi et al., 2000 A. Bernasconi N. Bernasconi Z. Caramanos D.C. Reutens F. Andermann F. Dubeau D. Tampieri B.G. Pike D.L. Arnold T2 relaxometry can lateralize mesial temporal lobe epilepsy in patients with normal MRI Neuroimage 12 2000 739 746 Briellmann et al., 2004a R.S. Briellmann G.D. Jackson G.S. Pell L.A. Mitchell D.F. Abbott Structural abnormalities remote from the seizure focus: a study using T2 relaxometry at 3 T Neurology 63 2004 2303 2308 Briellmann et al., 2004b R.S. Briellmann A. Syngeniotis S. Fleming R.M. Kalnins D.F. Abbott G.D. Jackson Increased anterior temporal lobe T2 times in cases of hippocampal sclerosis: a multi-echo T2 relaxometry study at 3 T AJNR Am. J. Neuroradiol. 25 2004 389 394 Cascino et al., 1991 G.D. Cascino C.R. Jack Jr. J.E. Parisi F.W. Sharbrough K.A. Hirschorn F.B. Meyer W.R. Marsh P.C. O’Brien Magnetic resonance imaging-based volume studies in temporal lobe epilepsy: pathological correlations Ann. Neurol. 30 1991 31 36 Duncan et al., 1996 J.S. Duncan P. Bartlett G.J. Barker Technique for measuring hippocampal T2 relaxation time AJNR Am. J. Neuroradiol. 17 1996 1805 1810 Duncan et al., 1997 J.S. Duncan P. Bartlett G.J. Barker Measurement of hippocampal T2 in epilepsy AJNR Am. J. Neuroradiol. 18 1997 1791 1792 Grunewald et al., 1994 R.A. Grunewald G.D. Jackson A. Connelly J.S. Duncan MR detection of hippocampal disease in epilepsy: factors influencing T2 relaxation time AJNR Am. J. Neuroradiol. 15 1994 1149 1156 Jack, 1996 C.R. Jack Jr. Hippocampal T2 relaxometry in epilepsy: past, present, and future AJNR Am. J. Neuroradiol. 17 1996 1811 1814 Jackson et al., 1993a G.D. Jackson S.F. Berkovic J.S. Duncan A. Connelly Optimizing the diagnosis of hippocampal sclerosis using MR imaging AJNR Am. J. Neuroradiol. 14 1993 753 762 Jackson et al., 1990 G.D. Jackson S.F. Berkovic B.M. Tress R.M. Kalnins G.C. Fabinyi P.F. Bladin Hippocampal sclerosis can be reliably detected by magnetic resonance imaging Neurology 40 1990 1869 1875 Jackson et al., 1993b G.D. Jackson A. Connelly J.S. Duncan R.A. Grunewald D.G. Gadian Detection of hippocampal pathology in intractable partial epilepsy: increased sensitivity with quantitative magnetic resonance T2 relaxometry Neurology 43 1993 1793 1799 Kiebel et al., 1999 S.J. Kiebel J.B. Poline K.J. Friston A.P. Holmes K.J. Worsley Robust smoothness estimation in statistical parametric maps using standardized residuals from the general linear model Neuroimage 10 1999 756 766 Meiners et al., 1994 L.C. Meiners A. van Gils G.H. Jansen G. de Kort T.D. Witkamp L.M. Ramos J. Valk R.M. Debets A.C. van Huffelen C.W. van Veelen Temporal lobe epilepsy: the various MR appearances of histologically proven mesial temporal sclerosis AJNR Am. J. Neuroradiol. 15 1994 1547 1555 Mueller et al., 2004 S.G. Mueller K.D. Laxer N. Cashdollar D.L. Flenniken G.B. Matson M.W. Weiner Identification of abnormal neuronal metabolism outside the seizure focus in temporal lobe epilepsy Epilepsia 45 2004 355 366 Mueller et al., 2007 S.G. Mueller K.D. Laxer N. Schuff M.W. Weiner Voxel-based T2 relaxation rate measurements in temporal lobe epilepsy (TLE) with and without mesial temporal sclerosis Epilepsia 48 2007 220 228 Pell et al., 2004 G.S. Pell R.S. Briellmann A.B. Waites D.F. Abbott G.D. Jackson Voxel-based relaxometry: a new approach for analysis of T2 relaxometry changes in epilepsy Neuroimage 21 2004 707 713 Pell et al., 2006a G.S. Pell R.S. Briellmann A.B. Waites D.F. Abbott D.P. Lewis G.D. Jackson Optimized clinical T2 relaxometry with a standard CPMG sequence J. Magn. Reson. Imaging 23 2006 248 252 Pell et al., 2006b G.S.H.P. Pell R.S. Briellmann D.F. Abbott G.D. Jackson Optimization of voxel based relaxometry parameters for the detection of focal T2 changes Proceedings of the International Society for Magnetic Resonance in Medicine Seattle, WA 2006 Peran et al., 2007 P. Peran G. Hagberg G. Luccichenti A. Cherubini V. Brainovich P. Celsis C. Caltagirone U. Sabatini Voxel-based analysis of R2* maps in the healthy human brain J. Magn. Reson. Imaging 26 2007 1413 1420 Rugg-Gunn et al., 2005 F.J. Rugg-Gunn P.A. Boulby M.R. Symms G.J. Barker J.S. Duncan Whole-brain T2 mapping demonstrates occult abnormalities in focal epilepsy Neurology 64 2005 318 325 Stafstrom, 2006 C.E. Stafstrom Epilepsy: a review of selected clinical syndromes and advances in basic science J. Cereb. Blood Flow Metab. 26 2006 983 1004 Townsend et al., 2004 T.N. Townsend N. Bernasconi G.B. Pike A. Bernasconi Quantitative analysis of temporal lobe white matter T2 relaxation time in temporal lobe epilepsy Neuroimage 23 2004 318 324 Van Paesschen et al., 1997 W. Van Paesschen A. Connelly M.D. King G.D. Jackson J.S. Duncan The spectrum of hippocampal sclerosis: a quantitative magnetic resonance imaging study Ann. Neurol. 41 1997 41 51 Worsley, 2003 Worsley, K.J., 2003. Developments in Random Field Theory. Human Brain Function, 2nd ed. (Online Version, Chapter 15).
更多查看译文
关键词
Hippocampal sclerosis,Partial seizures,Temporal lobe epilepsy,Epilepsy,MRI,Relaxometry
AI 理解论文
溯源树
样例
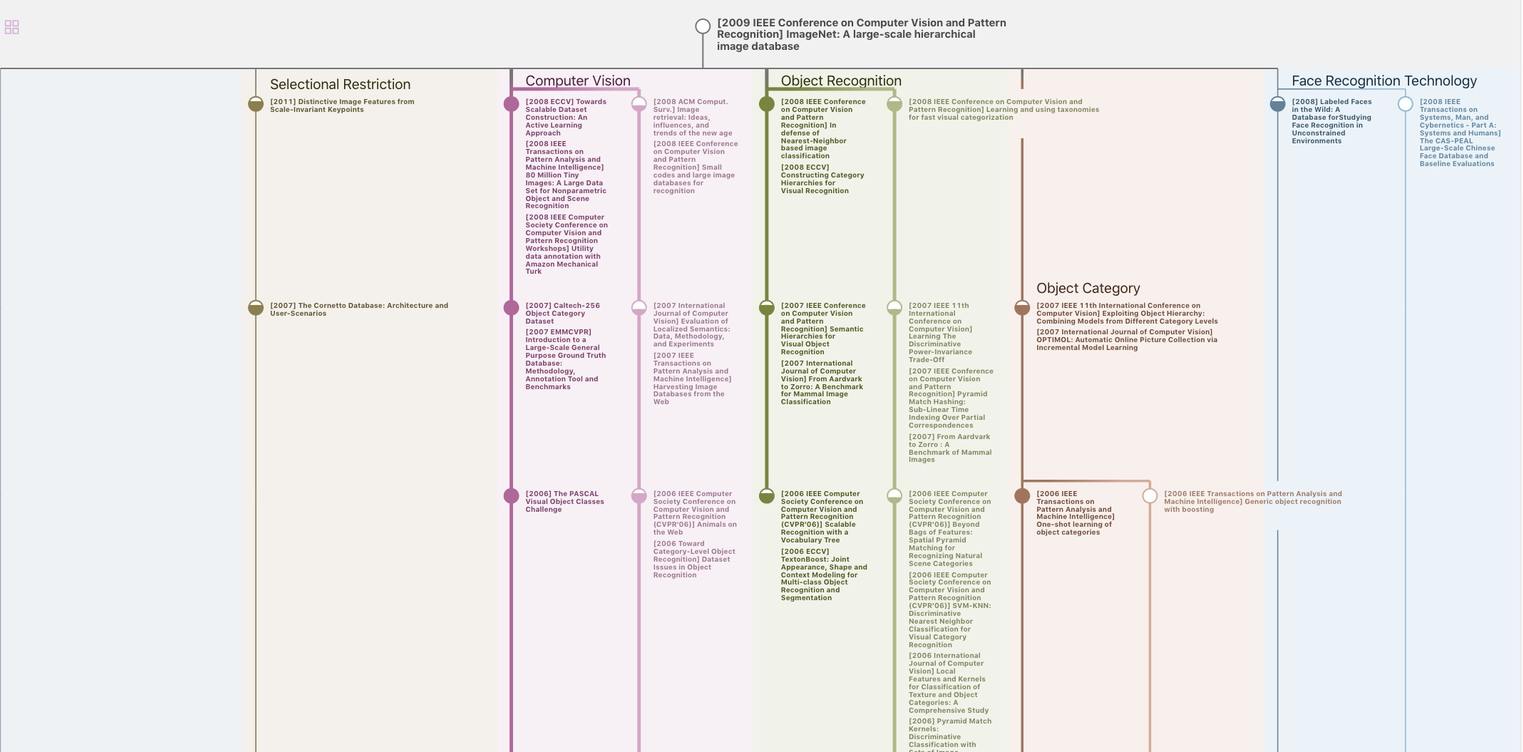
生成溯源树,研究论文发展脉络
Chat Paper
正在生成论文摘要