Reaction of human myoglobin and H2O2
msra(2001)
摘要
The sequence of human myoglobin (Mb) is similar to that of other species except for a unique cysteine at position 110 (Cys110). Adding hydrogen peroxide (H2O2) to human Mb affords Trp14-peroxyl, Tyr103-phenoxyl, and Cys110-thiyl radicals and coupling of Cys110-thiyl radicals yields a homodimer through intermolecular disulfide bond formation (Witting, P. K., Douglas, D. J., and Mauk, A. G. (2000) J. Biol. Chem.275, 20391–20398). Treating a solution of wild type Mb and H2O2 with 5,5-dimethyl-1-pyrroline-N-oxide (DMPO) at DMPO:protein ≤ 10 mol/mol yields DMPO-Cys110 adducts as determined by EPR. At DMPO:protein ratios (25–50 mol/mol), both DMPO-Tyr103 and DMPO-Cys110 adducts were detected, whereas at DMPO:protein ≥ 100 mol/mol only DMPO-Tyr103 radicals were present. The DMPO-dependent decrease in DMPO-Cys110 was matched by a near 1:1 stoichiometric increase in DMPO-Tyr103. In contrast, reaction of the Y103F human Mb with H2O2 gave no DMPO-Cys110 at DMPO:protein ≤ 10 mol/mol, and only trace DMPO-Cys110at DMPO:protein ≥ 100 mol/mol (i.e. conditions that consistently gave DMPO-Tyr103 in the case of wild type Mb). No detectable homodimer was formed by incubation of the Y103F variant with H2O2. However, the homodimer was detected in a mixture of both the Y103F and C110A variants of human Mb upon treatment with H2O2(C110A:Y103F:H2O2 2:1:5 mol/mol/mol); the yield of this homodimer increased with increasing ratios of C110A:Y103F. Together, these data suggest that addition of H2O2 to human Mb can produce Cys110-thiyl radicals through an intermolecular electron transfer reaction from Cys110 to a Tyr103-phenoxyl radical. The sequence of human myoglobin (Mb) is similar to that of other species except for a unique cysteine at position 110 (Cys110). Adding hydrogen peroxide (H2O2) to human Mb affords Trp14-peroxyl, Tyr103-phenoxyl, and Cys110-thiyl radicals and coupling of Cys110-thiyl radicals yields a homodimer through intermolecular disulfide bond formation (Witting, P. K., Douglas, D. J., and Mauk, A. G. (2000) J. Biol. Chem.275, 20391–20398). Treating a solution of wild type Mb and H2O2 with 5,5-dimethyl-1-pyrroline-N-oxide (DMPO) at DMPO:protein ≤ 10 mol/mol yields DMPO-Cys110 adducts as determined by EPR. At DMPO:protein ratios (25–50 mol/mol), both DMPO-Tyr103 and DMPO-Cys110 adducts were detected, whereas at DMPO:protein ≥ 100 mol/mol only DMPO-Tyr103 radicals were present. The DMPO-dependent decrease in DMPO-Cys110 was matched by a near 1:1 stoichiometric increase in DMPO-Tyr103. In contrast, reaction of the Y103F human Mb with H2O2 gave no DMPO-Cys110 at DMPO:protein ≤ 10 mol/mol, and only trace DMPO-Cys110at DMPO:protein ≥ 100 mol/mol (i.e. conditions that consistently gave DMPO-Tyr103 in the case of wild type Mb). No detectable homodimer was formed by incubation of the Y103F variant with H2O2. However, the homodimer was detected in a mixture of both the Y103F and C110A variants of human Mb upon treatment with H2O2(C110A:Y103F:H2O2 2:1:5 mol/mol/mol); the yield of this homodimer increased with increasing ratios of C110A:Y103F. Together, these data suggest that addition of H2O2 to human Mb can produce Cys110-thiyl radicals through an intermolecular electron transfer reaction from Cys110 to a Tyr103-phenoxyl radical. myoglobin 5,5-dimethyl-1-pyrroline N-oxide DMPO adduct of cysteine 110 DMPO adduct of tyrosine 103 diethylenetriaminepentaacetic acid protein radicals hydrogen peroxide 2-methyl-nitrosopropane millitesla metmyoglobin polyacrylamide gel electrophoresis Protein-centered free radicals are now recognized as intermediates for an increasing number of enzymes (1Stubbe J. van der Donk W.A. Chem. Rev. 1998; 98: 705-762Crossref PubMed Scopus (1368) Google Scholar). Examples include the thiyl (2Licht S. Gerfen G.J. Stubbe J. Science. 1996; 271: 477-481Crossref PubMed Scopus (283) Google Scholar) and tyrosyl (3Stubbe J. Biochemistry. 1988; 27: 3893-3900Crossref PubMed Scopus (79) Google Scholar) radical centers of ribonucleotide reductase, the tyrosyl radical of prostaglandin H synthase (4Lassmann G. Odenwaller R. Curtis J.F. DeGray J.A. Mason R.P. Marnett L.J. Eling T.E. J. Biol. Chem. 1991; 266 (; Correction (1992), J. Biol. Chem.267, 6449): 20045-20055Abstract Full Text PDF PubMed Google Scholar), the glycyl radical of pyruvate formate-lyase (5Wagner A.F. Frey M. Neugebauer F.A. Schafer W. Knappe J. Proc. Natl. Acad. Sci. U. S. A. 1992; 89: 996-1000Crossref PubMed Scopus (306) Google Scholar), and the tryptophanyl radical of cytochromec peroxidase (6Sivaraja M. Goodin D.B. Smith M. Hoffman B.M. Science. 1989; 245: 738-740Crossref PubMed Scopus (476) Google Scholar). Protein-centered radicals have also been implicated in oxidative stress associated with pathology of inflammatory disease(s) such as atherosclerosis and possibly in aging (7Dean R.T. Fu S. Stocker R. Davies M.J. Biochem. J. 1997; 324: 1-18Crossref PubMed Scopus (1470) Google Scholar, 8Halliwell B. Gutteridge J.M.C. Free Radicals in Biology and Medicine. 2nd Ed. Clarendon Press, Oxford1992Google Scholar). At least some of the toxic effects of protein radicals, as exemplified by protein-mediated initiation of lipid peroxidation (9Witting P.K. Willhite C.A. Davies M.J. Stocker R. Chem. Res. Toxicol. 1999; 12: 1173-1181Crossref PubMed Scopus (29) Google Scholar, 10Moore K.P. Holt S.G. Patel R.P. Svistunenko D.A. Zackert W. Goodier D. Reeder B.J. Clozel M. Anand R. Cooper C.E. Morrow J.D. Wilson M.T. Darley-Usmar V. Roberts 2nd, L.J. J. Biol. Chem. 1998; 273: 31731-31737Abstract Full Text Full Text PDF PubMed Scopus (244) Google Scholar, 11Miller Y.I. Altamentova S.M. Shaklai N. Biochemistry. 1997; 36: 12189-12198Crossref PubMed Scopus (168) Google Scholar, 12Miller Y.I. Felikman Y. Shaklai N. Arch. Biochem. Biophys. 1996; 326: 252-260Crossref PubMed Scopus (73) Google Scholar), involve translocation of radical centers from one amino acid residue to another through both intramolecular (13Wilks A. Ortiz de Montellano P.R. J. Biol. Chem. 1992; 267: 8827-8833Abstract Full Text PDF PubMed Google Scholar) and intermolecular (14Rao S.I. Wilks A. Ortiz de Montellano P.R. J. Biol. Chem. 1993; 268: 803-809Abstract Full Text PDF PubMed Google Scholar) mechanisms. Also, it has been proposed that free radical damage in proteins may well occur with a chain process similar to that for lipid peroxidation (15Neuzil J. Gebicki J.M. Stocker R. Biochem. J. 1993; 293: 601-606Crossref PubMed Scopus (146) Google Scholar). One example of radical translocation is provided by the protein-centered radical(s) formed upon reaction of mammalian myoglobin with H2O2 (16Lloyd-Raven E. Mauk A.G. Sykes A.G. Advances in Inorganic Chemistry: Heme-Fe Proteins. 51. Academic Press, London2000: 1-49Google Scholar). Myoglobin (Mb)1 shows a limited peroxidase activity in the presence of H2O2. Reaction of Mb and H2O2 yields water with the concomitant formation of ferryl (Fe(IV)=O) heme and a porphyrin radical cation (16Lloyd-Raven E. Mauk A.G. Sykes A.G. Advances in Inorganic Chemistry: Heme-Fe Proteins. 51. Academic Press, London2000: 1-49Google Scholar). At H2O2:protein ratios of ≤5, reaction of ferric or metmyoglobin (metMb) with H2O2yields both ferryl (Fe(IV)=O) Mb and protein radicals (globin⋅) (17George P. Irvine D.H. Biochem. J. 1952; 52: 511-517Crossref PubMed Scopus (202) Google Scholar). Although the ferryl Mb is stable for hours at room temperature and is characterized readily by electronic absorption spectroscopy (17George P. Irvine D.H. Biochem. J. 1952; 52: 511-517Crossref PubMed Scopus (202) Google Scholar), the identity of the Mb residue(s) that form globin⋅ in the presence of H2O2 has been the subject of some controversy. The precise location of the radical on the protein likely depends to some extent on both the mechanism by which it is formed and the stability of the radical species formed at a particular amino acid residue (14Rao S.I. Wilks A. Ortiz de Montellano P.R. J. Biol. Chem. 1993; 268: 803-809Abstract Full Text PDF PubMed Google Scholar). Globin⋅ have been localized to tyrosine (18Tew D. Ortiz de Montellano P.R. J. Biol. Chem. 1988; 263: 17880-17886Abstract Full Text PDF PubMed Google Scholar, 19Davies M.J. Biochim. Biophys. Acta. 1991; 1077: 86-90Crossref PubMed Scopus (142) Google Scholar) and/or tryptophan residues (20Gunther M.R. Tschirret-Guth R.A. Witkowska H.E. Fann Y.C. Barr D.P. Ortiz De Montellano P.R. Mason R.P. Biochem. J. 1998; 330: 1293-1299Crossref PubMed Scopus (134) Google Scholar) that effectively stabilize the odd electron through extended delocalization over unsaturated bonds. Additionally, globin⋅ may undergo subsequent chemistry (20Gunther M.R. Tschirret-Guth R.A. Witkowska H.E. Fann Y.C. Barr D.P. Ortiz De Montellano P.R. Mason R.P. Biochem. J. 1998; 330: 1293-1299Crossref PubMed Scopus (134) Google Scholar) and is capable of oxidizing other biological molecules (21Kelman D., J. DeGray J.A Mason R.P. J. Biol. Chem. 1994; 269: 7458-7463Abstract Full Text PDF PubMed Google Scholar). Importantly, hydroxyl radicals do not appear to be involved in the transfer of oxidative damage to either protein or target molecules (22Davies M.J. Free Radic. Res. Commun. 1990; 10: 361-370Crossref PubMed Scopus (105) Google Scholar, 23Turner J.J. Rice-Evans C.A. Davies M.J. Newman E.S. Biochem. J. 1991; 277: 833-837Crossref PubMed Scopus (75) Google Scholar). Human Mb is similar in sequence to other mammalian myoglobins. One significant difference, however, is the presence of Cys110 (24Hubbard S.R. Hendrickson W.A. Lambright D.G. Boxer S.G. J. Mol. Biol. 1990; 213: 215-218Crossref PubMed Scopus (79) Google Scholar). No other known mammalian Mb possesses a reactive thiol group. Recently (25Witting P.K. Douglas D.J. Mauk A.G. J. Biol. Chem. 2000; 275: 20391-20398Abstract Full Text Full Text PDF PubMed Scopus (71) Google Scholar), we investigated the reaction of human Mb and its C110A variant to evaluate the role of the reactive sulfhydryl group in the reaction of this protein with H2O2. Similar to sperm whale and horse heart Mb, reaction of human Mb and H2O2 yielded radicals derived from Trp14 and from tyrosine residues Tyr103 and Tyr146. Our data also indicated that the reaction of human Mb and H2O2 differed from the corresponding reaction of other myoglobin species by formation of Cys110-derived thiyl radical and that bimolecular coupling of thiyl radicals can lead to formation of a Mb homodimer through intermolecular disulfide bond formation. The spin trapping agent DMPO readily reacted with both Tyr103-phenoxyl and Cys110-thiyl radicals where present in reactions of human Mb and H2O2. However, the precise mechanism for the formation of the Cys110-thiyl radical on wild type human Mb was not elucidated. We now report further studies of the mechanism of Cys110-thiyl radical formation in the reaction of human Mb and H2O2 by the combined use of site-directed mutagenesis, gel electrophoresis, and EPR spectroscopy. Horse heart Mb, iodoacetamide, trypsin (Type III, 10, 200 unit/mg protein), urea, EDTA, 2,2,6,6-tetramethylpiperidine-N-oxyl (TEMPO), diethylenetriamine pentaacetic acid (DTPA), ammonium-d-camphor-10-sulfonate, 2-methyl-nitrosopropane (MNP), and DMPO were obtained from Sigma. DMPO was purified by stirring solutions (1 m in 50 mm phosphate buffer, pH 7.4) with activated charcoal (100 mg/ml) in the dark. After 30 min, the solution was filtered, and portions were stored at −80 °C prior to use (26Kotake Y. Reineke L.A. Tanigawa T. Koshida H. Free Radic. Biol. Med. 1994; 17: 215-223Crossref PubMed Scopus (34) Google Scholar). MNP was used without further purification. Solutions of MNP (500 mm in acetonitrile) were prepared immediately prior to use. Tryptone and yeast extract were from Becton Dickinson (Sparks, MD). Dithiothreitol and NaCl were obtained from Fisher Scientific (Fair Lawn, NJ). H2O2 was from Bio-Rad. Buffers were prepared from either glass distilled water or glass distilled water purified further by passage through a Barnstead Nanopure system. All buffers were stored over Chelex-100® (Bio-Rad) at 4 °C for at least 24 h to remove contaminating transition metals as verified by the ascorbate autoxidation analysis (27Buettner G.R. Methods Enzymol. 1990; 186: 125-127Crossref PubMed Scopus (77) Google Scholar). Organic solvents and all other chemicals employed were of the highest quality available. Restriction enzymes were from New England Biolabs or Life Technologies, Inc. DNA manipulations were performed using procedures described in Ref. 28Zoller M.J. Smith M. Methods Enzymol. 1987; 154: 329-350Crossref PubMed Scopus (129) Google Scholar. Complementary oligomers were synthesized, and DNA sequence analysis was performed at the UBC Nucleic Acid and Protein Services Unit (University of British Columbia, Canada). Site-directed mutagenesis was performed with the pBluescript II KS (±) vector (Stratagene, La Jolla, CA). DNA was amplified by the polymerase chain reaction with a high fidelity PfuTurbo® DNA polymerase (Stratagene). Point mutations were confirmed by DNA sequence analysis prior to protein expression in bacteria. Once the sequence was confirmed, the BamHI-HindIII fragment from the amplified DNA that also contained the mutant Mb coding was ligated to the BamHI-HindIII fragment from the vector pMb3 (29Ragavan V. Szabo A. Boxer S.G. Proc. Natl. Acad. Sci. U. S. A. 1985; 82: 5681-5684Crossref PubMed Scopus (98) Google Scholar) to yield the final expression vector. The circular plasmid was then transformed to the appropriate cell line for protein expression. TransformedEscherichia coli (strain AR68) containing plasmids for both the wild type recombinant human myoglobin and the C110A variant (29Ragavan V. Szabo A. Boxer S.G. Proc. Natl. Acad. Sci. U. S. A. 1985; 82: 5681-5684Crossref PubMed Scopus (98) Google Scholar) were obtained from Prof. S. G. Boxer. The DNA bearing the Y103F mutation was obtained by site-directed mutagenesis (above) and was used to transform E. coli DHB10 cells. For transformed bacteria (strain AR68), cells were grown at 28 °C in 10 × 2-liter flasks containing 2YT superbroth (1-liter flask: 16 g/liter tryptone, 10 g/liter yeast extract, and 5 g/liter NaCl) to an optical density (A600 nm) of 1.2–1.5. The expression of recombinant Mbs was induced by immersing each flask into a water bath (55 °C) for 5 min and then transferring the flask to an incubator operating at 42 °C. However, for the case of transformed DHB10 cells, cultures were grown at 28 °C in 10 × 2–1iter flasks containing 2YT superbroth to A600 = ∼3–4, and then the heat shock was applied in a similar fashion to that described for transformed AR68 cells. All cultures were subsequently incubated for a further 6–7 h, and the cells were harvested. Rapidly increasing the culture temperature in this manner was essential as simply increasing incubation temperature from 28 to 42 °C failed to induce protein expression. Myoglobin expressed by either cell line (isolated as a fusion product) was purified by anion exchange chromatography (Whatman DE52 resin) as described (29Ragavan V. Szabo A. Boxer S.G. Proc. Natl. Acad. Sci. U. S. A. 1985; 82: 5681-5684Crossref PubMed Scopus (98) Google Scholar). The partially purified fusion protein(s) was reconstituted with excess hemin (heme:protein = ∼1.5) (Porphyrin Products, Logan, UT), treated with trypsin (final concentration, ∼0.1 μg/ml) to cleave the fusion segment and then further purified as described (29Ragavan V. Szabo A. Boxer S.G. Proc. Natl. Acad. Sci. U. S. A. 1985; 82: 5681-5684Crossref PubMed Scopus (98) Google Scholar). Under these conditions, recombinant myoglobin was isolated as metMb. When required, proteins were concentrated by centrifugal ultrafiltration (Centriprep-10 concentrators, Millipore). The yield of recombinant protein obtained from E. coli DH10B cells was significantly lower than for the original cell line used to express human Mb; however, we were forced to use the former because of unavailability of the Ar68 cells. As a result of the poor yields (≤2 mg of protein/liter of culture medium), several protein samples were pooled to obtain sufficient material for our various studies. X-band EPR spectra (293 or 77 K) were obtained with a Bruker ESP 300e spectrometer equipped with a Hewlett Packard microwave frequency counter. Mb solutions (∼0.5 mm in 50 mm sodium phosphate buffer, pH 7.4) were treated with H2O2(H2O2:protein = ∼1.2–5 mol/mol) in both the presence and absence of the appropriate spin trap (spin trap:protein = 5–150 mol/mol). For spin trapping studies with MNP, the reagent was added from concentrated stock solutions to minimize effects of the organic solvent (final acetonitrile concentration in reaction mixtures, <5% v/v). Analyses of spin adducts were performed using samples (50 μl) of the reaction mixture transferred into capillary tubes with a glass pipette. The capillary was then placed into a quartz EPR tube, and the tube was transferred to the cavity for EPR analysis at 293 K. The limit of detection of a stable nitroxide (TEMPO) under identical conditions was determined to be ∼50 nm. Unless indicated otherwise, the time between removal of the sample, transfer to the appropriate cell, and tuning the spectrometer was consistently <30 s. EPR spectra were obtained as an average of 3–5 scans with modulation frequency 100 kHz and sweep time of 84 s. Microwave power, modulation amplitude, and scan range used for each analysis varied appropriately as indicated in the legends to the figures. Hyperfine couplings were obtained by spectral simulation using the simplex algorithm (30Duling D.R. J. Mag. Res. 1994; 104B: 105-110Crossref Scopus (900) Google Scholar) provided in the WINSIM program, which is available at the NIEHS, National Institutes of Health web site. All hyperfine couplings are expressed in units of mT. Simulations were considered acceptable if they produced correlation factors of R > 0.85. Peak areas were determined by integration with standard WINEPR software (Bruker). Yields of the DMPO-Cys110 and DMPO-Tyr103 adducts were determined by peak area comparison with 5 μm TEMPO prepared in 50 mm phosphate buffer, pH 7.4, and measured under similar experimental conditions. DTPA (100 μm) was included in all Mb solutions prior to the addition of H2O2 to minimize the possibility of free transition metal-mediated decomposition of peroxide by Fenton chemistry. CD spectroscopy was performed using a JASCO model J-720 spectropolarimeter calibrated with ammonium-d-camphor-10-sulfonate as described (31Maurus R. Overall C.M. Bogumil R. Luo Y. Mauk A.G. Smith M. Brayer G.D. Biochim. Biophys. Acta. 1997; 1341: 1-13Crossref PubMed Scopus (103) Google Scholar). Spectra (190–250 nm) were recorded for samples placed in a water-jacketed, cylindrical quartz cuvette (path length, 0.1 cm), and temperature was controlled with a NESLAB model RT 100 circulating water bath operated under computer control. The cuvette temperature was measured using a NESLAB RS-2 remote sensor interfaced into a computerized data acquisition system. Protein solutions (5–10 μm) were prepared in sodium phosphate buffer (10 mm, pH 7 and 8). Thermal denaturation curves were determined by monitoring ellipticity at 222 nm as temperature was increased from 25 to 85 °C (50 °C/h). Melting temperatures (Tm) are reported as the means ± S.D. from three measurements. Melting curves were transformed to ASCI text files for export to Scientist software (MicroMath, Salt Lake City, UT), which was used to calculate the first derivative of the curve for more accurate determination ofTm. The irreversibility of myoglobin folding under these conditions precluded determination of thermodynamic parameters consistent with a previous study using horse heart Mb (31Maurus R. Overall C.M. Bogumil R. Luo Y. Mauk A.G. Smith M. Brayer G.D. Biochim. Biophys. Acta. 1997; 1341: 1-13Crossref PubMed Scopus (103) Google Scholar). Electronic spectra were obtained with a Cary 3E UV/Vis spectrophotometer. Mb solutions were prepared in sodium phosphate buffer (50 mm, pH 7.4), and Mb concentration was determined spectrophotometrically (ε408 nm = 188,000 m−1cm−1) (32Antonini E. Brunori M. Hemoglobin and Myoglobin in Their Reaction with Ligands. North Holland Publishing Company, Amsterdam1971: 40-54Google Scholar). The pH-linked spectroscopic transitions of metMb were monitored between 280 and 700 nm from pH 6.3 to 10.5, and the corresponding pKa values were determined by fitting absorbance data obtained at 580 nm to the Henderson-Hasselbach equation with the program Scientist (MicroMath). Cross-linking experiments were performed by incubating solutions of either wild type human Mb or mixtures of the C110A and Y103F variants (protein concentration, 0.025–1 mm) with H2O2 (each in 50 mm sodium phosphate buffer, pH 7.4) at a protein:H2O2 ratio of ∼5 mol/mol and 37 °C. All samples contained the iron chelator DTPA (100 μm) to minimize the possibility of Fenton-type chemistry. After 30 min, the reaction mixture was diluted with 50 mmsodium phosphate buffer, pH 7.4 (final protein concentration, 25 μm), and each of the carefully matched samples was diluted further with nonreducing loading buffer (protein solution:loading buffer = ∼2 v/v). Nonreducing loading buffer was prepared by mixing 7 ml of 0.5 m Tris-Cl containing 0.4% SDS, 3.6 ml of glycerol, 1 g of SDS, and 1.2 mg of bromphenol blue, and finally water was added to 10 ml of final volume. Note that loading buffer did not contain either mercaptoethanol or dithiothreitol because these reagents can reduce the dimeric product (25Witting P.K. Douglas D.J. Mauk A.G. J. Biol. Chem. 2000; 275: 20391-20398Abstract Full Text Full Text PDF PubMed Scopus (71) Google Scholar). Dilute protein samples were then heated at 90–100 °C (5 min) and analyzed by SDS-PAGE (33Laemmli U.K. Nature. 1970; 227: 680-685Crossref PubMed Scopus (207537) Google Scholar) after staining with Coomassie Blue. Statistics were performed with Student's t test available in MS Excel (Microsoft), and significant difference was accepted at p < 0.05. To investigate the relationship between Tyr103-phenoxyl radicals and the formation of the Cys110-thiyl radical, we sought to prepare the Y103F variant of human Mb. It has been reported previously that the point mutation Y103F in recombinant sperm whale myoglobin destabilizes the protein sufficiently that isolation of the variant is precluded (13Wilks A. Ortiz de Montellano P.R. J. Biol. Chem. 1992; 267: 8827-8833Abstract Full Text PDF PubMed Google Scholar). For this species of Mb, the destabilizing influence of the Y103F substitution can be offset by introduction of a second point mutation, K102Q (13Wilks A. Ortiz de Montellano P.R. J. Biol. Chem. 1992; 267: 8827-8833Abstract Full Text PDF PubMed Google Scholar). In contrast, the expression of the corresponding Y103F variant of human Mb afforded a stable protein despite the presence of Lys102 (24Hubbard S.R. Hendrickson W.A. Lambright D.G. Boxer S.G. J. Mol. Biol. 1990; 213: 215-218Crossref PubMed Scopus (79) Google Scholar) in the sequence for the human protein. In view of this difference in stability between these mammalian proteins to the alteration at Tyr103, we have further characterized the Y103F variant Mb by electronic absorption and CD spectroscopy and determined the pKa for titration of the water molecule coordinated to the ferric iron of the variant and wild type human proteins. The electronic spectrum obtained from solutions of the Y103F variant of human Mb exhibited Soret (409 nm) and visible bands (λmax= 505 and 631 nm) similar to those of the wild type protein (Fig.1 A), consistent with corresponding spectra reported for the wild type and Y103F/K102Q variant of sperm whale Mb (see Fig. 4 in Ref. 13Wilks A. Ortiz de Montellano P.R. J. Biol. Chem. 1992; 267: 8827-8833Abstract Full Text PDF PubMed Google Scholar). The CD spectra for wild type human Mb and the Y103F variant of human Mb (10 mmphosphate buffer, pH 8) were also virtually identical (Fig.1 B) and indicated that the two proteins possess similar helical content at pH 7 (not shown). The melting curves for each protein afforded similar Tm values at pH 8:Tm = 77.3 ± 0.5 and 76 ± 1 °C (data represent means ± S.D., n = 3) for wild type Mb and the Y103F variant, respectively (Fig. 1 B,inset). Melting curves determined for the two proteins at pH 7 (10 mm phosphate buffer) also afforded similarTm values: Tm = 80 ± 1 and 78.1 ± 0.2 °C for wild type human and Y103F variant proteins, respectively (not shown). These Tmvalues were not significantly different independent of the pH of the buffer employed as judged by t test analyses (p≫ 0.05). On the other hand, the pKa determined for the Y103F variant was significantly lower (p < 0.05) than that of the wild type protein (8.26 ± 0.09 and 8.85 ± 0.03, respectively, mean ± SD, n = 3) (Fig.1 A, inset). This difference in pKa indicates that the Y103F point mutation probably induces some subtle change in the heme binding site that alters the binding of the water ligand. The crystal structure of a related (K45R/C110A) variant of human Mb (24Hubbard S.R. Hendrickson W.A. Lambright D.G. Boxer S.G. J. Mol. Biol. 1990; 213: 215-218Crossref PubMed Scopus (79) Google Scholar) indicates that Tyr103resides near the surface of the protein and is the tyrosine residue nearest the heme group as is also the case for sperm whale Mb (34Takano T. J. Mol. Biol. 1977; 110: 537-568Crossref PubMed Scopus (613) Google Scholar). For the human protein, the point of closest approach is a phenyl carbon that is ∼3.44 Å from the edge of the heme group. Conceivably, a minor reorganization of the heme pocket that is not detected by electronic spectroscopy or by visible CD spectroscopy affords a lower pKa for the acid-base transition in the Y103F protein. Regardless of the basis for the difference in this pKa value, it is clear that the thermal stability and the electronic absorbance characteristics of the Y103F variant are comparable with that of the wild type protein. We next investigated the formation of globin⋅ in the reactions of wild type human Mb and both the C110A and Y103F variants with H2O2 using the spin traps DMPO and MNP. Addition of H2O2 to solutions of wild type human Mb containing DMPO at DMPO:protein ≤ 10 mol/mol consistently produced a product with an EPR spectrum comprised of a broad four-line signal (Fig.2 A, solid line). This broad signal was simulated well (Fig. 2 A, broken line) with hyperfine data previously reported for the trapping of thiyl radicals on human Mb at Cys110(DMPO-Cys110) (25Witting P.K. Douglas D.J. Mauk A.G. J. Biol. Chem. 2000; 275: 20391-20398Abstract Full Text Full Text PDF PubMed Scopus (71) Google Scholar). The treatment of the C110A variant of human Mb with peroxide in the presence of DMPO under identical conditions, however, consistently gave a more complex EPR spectrum (Fig. 2 B, solid line). This complex signal was also detected in the corresponding reaction of horse heart Mb and H2O2 (Fig. 2 C) where the radical has been assigned previously as resulting from the DMPO adduct of a Tyr103-phenoxyl radical (DMPO-Tyr103) (20Gunther M.R. Tschirret-Guth R.A. Witkowska H.E. Fann Y.C. Barr D.P. Ortiz De Montellano P.R. Mason R.P. Biochem. J. 1998; 330: 1293-1299Crossref PubMed Scopus (134) Google Scholar). Thus, the DMPO adduct derived from the reaction of C110A variant of human Mb and H2O2 was readily simulated (20Gunther M.R. Tschirret-Guth R.A. Witkowska H.E. Fann Y.C. Barr D.P. Ortiz De Montellano P.R. Mason R.P. Biochem. J. 1998; 330: 1293-1299Crossref PubMed Scopus (134) Google Scholar) with the reported coupling values (Fig. 2 B, broken line), and the globin⋅ was assigned as a DMPO-Tyr103 adduct. These data support the conclusion that Cys110-thiyl radicals are thermodynamically stable end products following reaction of the wild type protein with hydrogen peroxide, whereas for Mb lacking Cys110, the Tyr103-phenoxyl radical is the most stable globin⋅produced. In marked contrast to the analogous reaction with wild type human Mb, no radical adducts were detected upon addition of H2O2 to a solutions containing the Y103F variant and DMPO (Y103F:H2O2:DMPO 1:5:≤10 mol/mol/mol) (Fig. 3 A). Furthermore, at the highest trap efficiency investigated (DMPO:H2O2:Y103F 1:5:∼100 mol/mol/mol), only a weak four-line EPR spectrum was obtained, and this spectrum exhibited hyperfine coupling identical to that for the DMPO adduct derived from trapping DMPO-Cys110 radicals (Fig. 3 B). Under these conditions, the corresponding reaction of wild type human Mb and H2O2 gave both DMPO-Cys110 and DMPO-Tyr103 adducts (e.g., see Fig. 2). Overall, the concentrations of DMPO-Cys110 radicals measured from the wild type and Y103F variant proteins varied by ∼130-fold as determined by double integration of the respective DMPO-Cys110 EPR signals from the two proteins. Collectively, these data independently confirm the alteration at Tyr103 in the Y013F variant. Radical adducts of protein-derived tyrosine phenoxyl radicals have also been reported to form with the nitroso spin trap MNP (20Gunther M.R. Tschirret-Guth R.A. Witkowska H.E. Fann Y.C. Barr D.P. Ortiz De Montellano P.R. Mason R.P. Biochem. J. 1998; 330: 1293-1299Crossref PubMed Scopus (134) Google Scholar, 35Fenwick C.W. English A.M. J. Am. Chem. Soc. 1996; 118: 12236-12237Crossref Scopus (65) Google Scholar, 36Barr D.P. Gunther M.R. Deterding L.J. Tomer K.B. Mason R.P. J. Biol. Chem. 1996; 271: 15498-15503Abstract Full Text Full Text PDF PubMed Scopus (127) Google Scholar). Therefore, to verify that replacement of Tyr103 resulted in the loss of the Tyr103-phenoxyl radical, MNP was employed in reactions of both the wild type and Y103F variant Mb with H2O2 (Mb:H2O2:MNP 1:5:20 mol/mol/mol). For the case of wild type human Mb, reaction mixtures treated with MNP afforded a radical with a three-line EPR spectrum after 2 min at 20 °C (Fig
更多查看译文
AI 理解论文
溯源树
样例
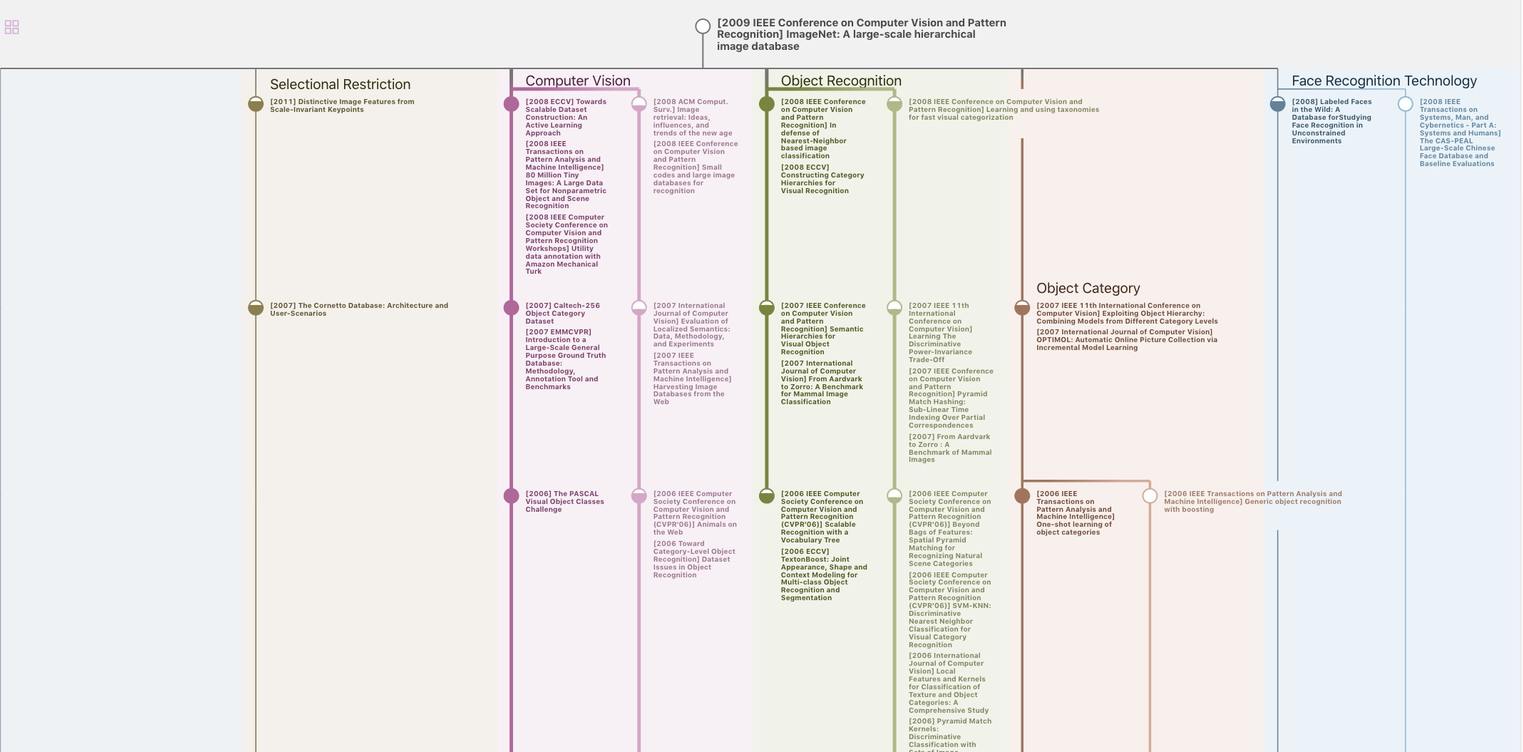
生成溯源树,研究论文发展脉络
Chat Paper
正在生成论文摘要