Geochemical and isotopic evidence for palaeo-seawater intrusion into the south coast aquifer of Laizhou Bay, China
Applied Geochemistry(2011)
摘要
This research aims to improve the current knowledge of groundwater salinisation processes in coastal aquifers using combined hydrochemical and isotopic parameters and inverse hydrochemical modelling. Field investigations were conducted in Laizhou Bay, which is the area most seriously affected by seawater intrusion in north China. During three sampling campaigns along a vertical transect in the Changyi-Liutuan area, 95 ground- and surface-water samples were collected for major ion and isotope analysis ( 2 H/ 18 O, 3 H, 14 C, 34 S). The groundwater changes along the general flowpath towards the coast from fresh (<1 g/L), brackish (1–10 g/L), saline (10–100 g/L) to brine water (>100 g/L). Molar Cl/Br ratios are close to those of seawater in almost all groundwater samples, indicating that brines and deep seawater evolved from different events of palaeo-seawater intrusion. Depleted isotopic signatures of brines and deep saline water point to a former, initially depleted seawater reservoir due to runoff dilution. Tritium and 14 C activities in deep saline water below confining units indicate isolation from modern precipitation and significant residence times. Brine water shows a wide range of 3 H and 14 C ages due to the complex conditions of mixing without isolation from modern groundwater. Sulphur-34 isotope ratios support seawater intrusion as a possible salt origin, although this parameter does not exclude gypsum dissolution. The combined use of Cl and 18 O yields four different end-members of groundwater, and three different mixing scenarios were identified explaining the hydrochemical composition of groundwater samples with intermediate salinity in the different areas. To improve understanding of the various water types and their related processes in a spatial context, a conceptual model was developed integrating the results derived from the presented data in a vertical cross-section. Results of three inverse modelling simulations using PHREEQC-2 show that all hypothetical mixing scenarios derived from conservative components are thermodynamically feasible. In all scenarios, mixing, ion exchange, dissolution of dolomite and precipitation of gypsum and calcite account for the hydrochemical changes. 1 Introduction Declining groundwater levels, caused by continuous over-exploitation of coastal aquifers, can result in saline water intrusion ( Pinder, 1973; Custodio and Bruggeman, 1987; Essaid, 1990; Jones et al., 1999; Pulido-Leboeuf, 2004; Grassi et al., 2007 ). The associated increase in concentrations of total dissolved solids (TDS) can eventually render freshwater resources unusable ( Sherif and Hamza, 2001 ). Saline water intrusion threatens drinking water supplies, a large proportion of which are derived from groundwater, in the coastal region of China. The Bohai Sea area, especially Laizhou Bay, experiences the most severe seawater intrusion in North China ( Fig. 1 a) ( Meng et al., 1997 ). Concerns for long-term human and environmental health make it important to understand how geological conditions control flow dynamics in coastal aquifers and how water and chemical fluxes change in the freshwater–saltwater interface. The combination of chemical and isotopic indicators to characterise the behaviour of salty water in the coastal groundwater circulation has previously been applied to determine the origins of groundwater salinity, delineating flow systems and groundwater salinisation processes, examine migration of the fresh–salt water interface and understand the mixing relationships between saline water bodies and surrounding freshwater in many coastal aquifers (e.g., Bergelson et al., 1999; Kim et al., 2003; Marimuthu et al., 2005; Schiavo et al., 2009 ). To successfully describe groundwater flow, transport, and geochemical interactions under variable density conditions in relation to real-world problems, detailed and accurate characterisation of the subsurface is required. Increasing Cl − and TDS concentrations towards the coastline are good indicators of seawater intrusion. In the process of seawater intrusion, mixing between saline and freshwater and water–rock interaction (e.g., cation exchange processes with clays) may influence groundwater salinity ( Appelo and Postma, 2005; Vengosh et al., 2002; Sivan et al., 2005; Edmunds and Smedley, 2000; Grassi and Cortecci, 2005; Möller et al., 2007 ). Using multiple sources of chemical and isotope data combined with hydrogeological information may significantly improve the current understanding of seawater intrusion processes. This paper presents a case study from the south coast plain of Laizhou Bay, Shandong province, north China. Previous work has largely dealt with saltwater intrusion mechanisms, groundwater flow modelling, the formation of brine waters, and preventive countermeasures for seawater intrusion (e.g., Zhang et al., 1996; Li et al., 2000 ), which has become the critical focus of hydrogeological research in the bay (e.g., Chen et al., 1997; Zhang and Peng, 1998; Xue et al., 2000 ). The management of this coastal aquifer requires an understanding of the main processes controlling the groundwater salinity and geochemical evolution. In contrast to previous work, this study integrates 2 H/ 18 O data and 3 H, 34 S and 14 C analyses. A number of samples from the deeper aquifers are included, giving additional insight into the hydrogeochemical processes at different depths. Based on this extensive data set, this research aims to demonstrate how major ion chemistry, isotopes, hydraulic data and hydrochemical modelling can be combined in a multi-approach study related to saltwater intrusion to (i) identify the different salt sources and their contribution to the present horizontal and vertical salinity distribution, (ii) estimate the groundwater age and mixing behaviour between different end-members, and (iii) improve the understanding of reactive transport processes in determining the hydrogeochemical evolution of different types of groundwater. 2 Study area 2.1 Geological and hydrogeological setting The southern part of Laizhou Bay covers approximately 2870 km 2 in Shandong province, northeastern China ( Fig. 1 a). The northern boundary of the study area is Laizhou Bay (Bohai Sea) with a coastline of 12.8 km. It contains a series of Cretaceous to Recent sediments that overlie the Palaeozoic basement. The depositional facies of the aquifer change from south to north, from alluvium to proluvial to marine sediments on the coastal plain. The main aquifers in the study area are composed of Quaternary sediments with a thickness of 30–50 m in the south and up to 300 m in the north ( Xue et al., 2000 ). The strata in the upper proluvial fan in the south mainly consist of gravel and coarse sandstone, grading to fine sand, silt, sandy clay, and silty clay towards the coast. The vertical structure is shown in Fig. 1 b. The northern part of the aquifer in the study area is split into two different units separated by confining layers. There is a general upwards trend from coarse- to fine-grained material. As shown in Fig. 1 b, the southern mountains and the unconfined part of the aquifer constitute the main recharge areas, and the general natural groundwater flow pattern is from south to north although the general flow pattern may be altered considerably by highly permeable erosion channels crossing the area, as shown in the cross-section ( Fig. 1 b). Brines have been detected up to 10 km from the shoreline ( Han et al., 1996; Zhang and Peng, 1998 ). According to previous studies by Xue et al. (2000) the brines were assumed to have originated from ancient seawater during three occurrences of marine invasion and regression along the coast of Laizhou Bay since the middle Late Pleistocene (39–24 ka B.P.), and formed by evaporation of ancient seawater in combination with re-dissolution of salts and mixing. Brine water is mainly distributed in depths of 0–15 m, 33–42 m, and 59–74 m, forming three different layers separated by confining units. The mineralogy of the shallow aquifer includes quartz, anorthite, albite, plagioclase feldspar, picrite, biotite, aragonite, dolomite, calcite, kaolinite, gibbsite and Ca–montmorillonite. Only minor amounts of evaporites (e.g., gypsum, anhydrite, halite, polyhalite, bischofite, epsomite) are present locally ( Zhang et al., 1996; Han et al., 1996; Xue et al., 2000 ). Owing to the marine depositional environment, there are more chemical sediments in the aquifer matrix towards the coastline, especially in the saline and brine water zones ( Han et al., 1996 ). Gypsum deposits are common in the coastal saltwater zone ( Zhang and Peng, 1998 ). By contrast, silicate rocks dominate the freshwater zone at the top of the Wei River alluvial fan to the south. The clay layers are composed of illite, kaolinite and chlorite ( Zhao, 1996 ). In the southern mountainous areas of Laizhou Bay, bedrock is mainly composed of Cretaceous and Neogene basalt, andesite, trachyte and pyroclastic rock that consist of anorthite, albite, K-feldspar and biotite ( Ning, 2004 ). In the area near Xiaying, the lithology is fine sand, with coarse sand to gravel from the upper to the lower part, the main minerals being quartz, mica and feldspar. Calcite-rich nodules and re-precipitated gypsum occur in the clay interbed layer ( Han et al., 1996 ). Additionally, in the downstream region of Weihe River, a palaeochannel is well developed, with very thick layers of coarse-grained deposits formed during the early Late Pleistocene. The palaeochannel provides well-connected paths for brine/seawater intrusion ( Li et al., 2000 ). From south to north, the buried depth of the top of the sand layer in the alluvial fan ranges from 5 to 50 m; the depth to the bottom is 8–65 m. 2.2 Climatic and hydrological background On the basis of a 45-a record in the study area, the mean annual precipitation is estimated to be 630 mm, and the average annual temperature is 12 °C. Of the total annual precipitation, 60–70% falls between June and August. The average annual evaporation based on pan evaporation experiments is about 1640 mm, chiefly from April to June. Elevation ranges from 30 m a.s.l. in the south to 1–2 m a.s.l. in the north ( Chen et al., 1997 ). The Weihe River is the main water course in the study area and shows intermittent discharge characteristics, depending on rainfall. Its annual average streamflow is 1.5 × 10 8 m 3 /a. The river head is normally located several metres above the groundwater level, and infiltration from the river recharges the groundwater. 2.3 Environmental issues Owing to the concentration of human habitation, urbanisation and agricultural activity, increasing demand for groundwater has caused a gradual increase in saltwater intrusion over recent years, a problem that has been known to local authorities since 1976 ( Ji, 1991 ). Before 1975, the water demand was lower and the groundwater in the study area retained its natural status, flowing from south to north, then discharging into Laizhou Bay. After 1983, two water supply centres were installed that disrupted the natural flow pattern by forming two drawdown cones caused by over-exploitation under weak recharge conditions. The first cone near Changyi city, about 22 km away from the coast, has led to a water level decline of 15 m and the intrusion of saline water into the freshwater aquifer ( Fig. 1 a). The heavy over-exploitation of groundwater resources in the deep aquifers results in a downward gradient between the upper and the lower aquifers ( TJR, 2006 ). Nonetheless, the hydraulic head of the deep aquifer is locally higher than the shallower groundwater level, leading to upconing of the fresh–salt water interface (location indicated in the cross-section of Fig. 1 b). The second depression is located in the coastal aquifer of Laizhou Bay near Xiaying close to the coast and is related to exploitation of shallow brines for obtaining salt by means of evaporation since the late 1980s ( Chen et al., 1997 ). Here the groundwater level dropped to −22.7 m a.s.l. in 2005, showing an annual decline of up to 1 m/a. According to Fetter (1994) and Nonner (2006) , groundwater samples were divided into four types: fresh, brackish, saline and brine water, with TDS of <1 g/L, 1–10 g/L, 10–100 g/L, and >100 g/L, respectively. Under natural conditions before intensive groundwater exploitation, a gradual transition from brines to fresh water had developed over an extended period, leading to the formation of brackish and saline water as intermediate types. The excessive abstraction of fresh water caused the brackish and salt water to advance landwards, forming an undulating salt water/fresh water interface due to exploitation dynamics and the hydrogeological heterogeneity. 3 Methods Water was sampled at different aquifer depths during three periods from November 2005 to August 2007, along a 44.4 km cross-section in the Changyi-Liutuan area of Laizhou Bay ( Fig. 2 ). 58 groundwater wells ranging from 10 to 280 m depth ( Appendix – Table 1 ) were sampled throughout the Quaternary aquifer. Most of the groundwater samples were collected from irrigation and domestic supply wells, some of which were being continuously pumped. Some of the irrigation wells have long screened intervals (several metres), and, therefore, water samples are from a relatively wide section of the aquifer. Monitoring wells SG29, SG40, SG42, SG44, and SG47 ( Appendix – Table 1 ) have screens of less than 1 m in length. Shallow groundwater refers to pore water in the phreatic aquifer, with a depth of 6–60 m, and deep groundwater to water from the confined aquifer, with a depth of 60–280 m. Some surface-water samples (labelled SU) were also taken. Ninety-five samples were collected from screened intervals: 41 in November 2005, 25 in July 2006 and 29 in August 2007. Groundwater samples were divided into shallow groundwater (SG) and deep groundwater (DG) ( Appendix – Table 2 ). Groundwater was sampled by pumping after constant values of conductivity and redox potential had been established ( Fig. 2 ). The pH, temperature and conductivity were measured in the field using a portable WM-22EP meter. Surface- and groundwater samples were filtered immediately after sample retrieval for analysis of cations and anions using 0.45 μm membrane filters. Samples for cation analysis were preserved in acid-washed polyethylene bottles and acidified to pH < 2 with 6 N HNO 3 . In most cases the alkalinity of filtered samples was determined in the field by titration with H 2 SO 4 (0.22 N); otherwise alkalinity samples were collected in glass bottles which were carefully filled without any air entrapment for analysis in the laboratory. All collected samples were stored at 4 °C in HDPE bottles with watertight caps. Hydrochemical analyses were performed using a High Performance Ion Chromatograph (SHIMADZU). Only analyses with charge balance errors below 5% were considered in this study. Stable isotope values of δ 18 O and δ 2 H were measured by mass spectrometry, with a Finnigan MAT253 after on-line pyrolysis with a Thermo Finnigan TC/EA (Temperature Conversion Elemental Analyzer), in the Institute of Geographic Sciences and Natural Resources Research, Chinese Academy of Sciences. The δ 18 O and δ 2 H values were measured relative to internal standards that were calibrated using IAEA standards. Data were normalised following Coplen (1988) and are expressed in conventional δ (‰) notation. The analytical precision was ±2‰ for δ 2 H and ±0.3‰ for δ 18 O. The results are shown in Table 1 . The 3 H contents of water samples ( Table 2 ) were measured at the Institute of Hydrogeology and Environmental Geology, Chinese Academy of Geological Sciences. After distillation of 250 mL of the water sample, the samples were electrolytically enriched at 0.5 °C. Analysis was performed using a 1220 Quantulus ultra low level liquid scintillation spectrometer. The data were normalised and expressed using the method of Rozanski and Gröning (2004) . Results are reported in tritium units (TU), with a typical error of ±0.12 TU. The δ 13 C values of dissolved inorganic carbon (DIC) in water samples were measured using a Finnigan MAT 253 isotope ratio mass spectrometry (IRMS) at the Institute of Geographic Sciences and Natural Resources Research, Chinese Academy of Sciences. The Total Dissolved Inorganic C (TDIC) was separated in the field in the form of a precipitate of BaCO 3 . The resulting BaCO 3 samples were analysed for C isotope ratios as CO 2 gas, released by phosphoric acid treatment followed by dual inlet isotope ratio mass spectrometry. The δ 13 C DIC values of water samples ( Table 2 ) are reported in ‰ relative to V-PDB. Precision (1 σ ) for δ 13 C DIC is ±0.3‰. The 14 C activities were determined by a compact AMS system based on a NEC (National Electrostatics Corporation) Model 1.5SDH-1 Pelletron accelerator in the Institute of Heavy Ion Physics & Key Laboratory of Heavy Ion Physics, Ministry of Education, Peking University, Beijing. The activities are expressed as percent modern C (pmC) ( Table 2 ). The accuracy of this system is better than 0.4%, and the instrument background is lower than 0.03 pmC. By convention, the 14 C age is expressed in years BP (Before Present), where 1950 is taken as the start year. Calculations of minimum 14 C ages were carried out according to Clark and Fritz (1997) : (1) t = - 8267 · ln a t 14 C q · a o 14 C with t = groundwater age in years, a t 14 C = measured activity of 14 C, a 0 14 C = modern 14 C activity of soil derived DIC and q = mixing factor. The mixing factor accounts for the dissolution of calcite, which is assumed to be free of 14 C and, therefore, dilutes the initial 14 C activity of aqueous DIC in recharged water. Several methods exist to calculate the q factor, but the selection of an adequate approach for all the water samples is problematic owing to their different ages and conditions of recharge. An overview of the different methods is given by Clark and Fritz (1997) . Conditions during recharge in the study site are unknown and may have changed from rain water to seawater infiltration under open and/or closed conditions over space and time. Therefore, in this study different methods were used to correct 14 C ages for carbonate dissolution considering either only chemical mixing between the C compounds or isotopic fractionation using 14 C activities, δ 13 C values and alkalinities as compiled in Table 3 . The input variables and references used in the corresponding methods are compiled in Table 4 . 3.1 Sulphate isotopes The δ 34 S SO4 samples were collected in 1 L polyethylene bottles and acidified to pH < 3 with 6 N HNO 3 . The δ 34 S SO4 was determined by converting precipitated BaSO 4 to SO 2 using the method of Halas and Szaran (1999) . Analysis was conducted on a Finnigan MAT Delta-S gas mass spectrometer in the Laboratory for Stable Isotope Geochemistry, Institute of Geology and Geophysics, Chinese Academy of Sciences. The isotopic data are reported in δ (‰) notation relative to V-CDT (Canyon Diablo Troilite). Analytical precision for duplicates of samples and internal standards was better than ±0.4‰. The δ 34 S values are shown in Table 5 . 4 Results and discussion 4.1 Distribution of salinity and hydrogeochemistry The Stiffmap plot ( Fig. 3 ) indicates lateral zoning in groundwater hydrochemistry, with changes from fresh to brackish, to saline, to brine and then to saline and seawater from the south towards the coast, which is in agreement with the previous studies by Xue et al. (2000) . TDS contents range from 0.32 to 144.6 g/L, within the range previously reported for the region by Xue et al. (2000) . Sodium increases from 39% of total cations in the fresh waters with TDS concentrations <1 g/L to 71% in the saline waters with 10–100 g/L TDS concentrations, and up to 76% in brine waters. Correspondingly, Cl increases from 26% of total anions in fresh water to 88% in the saline water, and Ca decreases from 40% of total cations in fresh water to 4% in the saline water, and down to 2% in brine waters. Increasing salinities and the changing proportions of Na and Cl indicate evapoconcentration or halite dissolution as a major source of elevated TDS concentrations. Calcium–HCO 3 -type freshwater was found in most samples south of the drawdown cone ( Fig. 3 ), whereas brackish water ranging from Na–HCO 3 ·Cl type to Na–Cl·HCO 3 type to Na–Cl type is located mostly in the Changyi drawdown cone. Rising groundwater salinities in the groundwater depression are caused by the progression of seawater intrusion, by brines or by the upconing of saline deep groundwater, due to heavy exploitation. The greatest distance of brackish water away from the coastline is 25.4 km (sample SG29). However, within the drawdown cone freshwater was also found in some shallow groundwater samples such as SG23, SG40, SG42, SG43, SG45 and SG48 ( Fig. 2 ). These samples are from close to the upper part of the alluvial fan and the palaeochannel and form freshwater lenses not subjected to salinisation, probably owing to the heterogeneity of the aquifer. For instance, in all three sampling periods SG23 and SG40 showed freshwater composition at 20 and 14 m depth respectively, floating on a brackish wedge documented by SG48 at 30 m depth. Both samples SG23 and SG40 were of the Na–HCO 3 type, indicating that freshwater had replaced saltwater. In the northern and central part of the study area, confining units separate the sediments into a shallow and a deep aquifer ( Fig. 1 b). While brines dominate in the upper part, saline water was present below the confining unit. The brines have salinities up to 4.56 times higher than seawater (TDS > 125 g/L). Oversaturation of calcite and dolomite indicates the possibility of calcite and dolomite precipitation in saline and brine water. Gypsum reaches saturation and the possibility of precipitation only in brine samples, whereas all samples are undersaturated in halite ( Fig. 4 ). Saline water of the NaCl-type with TDS between 10 and 100 g/L was present only in the deep aquifer below and north of the drawdown cone ( Figs. 3 and 5 ). The relative cation and anion concentrations in the groundwater ( Fig. 5 ) indicate cation exchange reactions related to salinisation. Three hydrochemical end-members are evident in Figs. 3 and 5 : (i) freshwater, (ii) seawater and (iii) brines. Excess of Cl compared to Na (CaCl 2 type) was observed in the saline water and brines north of the depression, indicating exchange of Na for Ca desorbed from the mineral surfaces. This is a common process and occurs when saline water enters an aquifer that was previously in equilibrium with a less mineralised water of the Ca(HCO 3 ) 2 type. The reverse process was observed in brackish water of the drawdown cone, where NaHCO 3 waters point to exchange of Ca for Na desorbed from the mineral surfaces owing to freshwater entering an aquifer that was previously in equilibrium with more mineralised water of the Na–Cl type ( Figs. 3 and 5 ). The simultaneous occurrence of salinisation and freshening in different parts of the aquifers is attributed to changing groundwater levels and flow directions at a local scale as a result of changing rates of groundwater extraction. This leads to changes of the groundwater geochemistry by promoting ion exchange reactions. Seawater samples were sampled very close to the shoreline at shallow water depths below 1 m and therefore show considerable variations in Cl and SO 4 . Generally, the TDS concentration of surface water is lower than that of shallow and deep groundwater, ranging from 0.3 to 1.0 g/L (mean 0.5 g/L), with the hydrochemical type Ca·Na–HCO 3 ·Cl. This reflects the fact that surface water is mainly recharged by precipitation and surface runoff and not by groundwater as described above. Only SU10, being close to the coastline, is subjected to mixing with seawater. Temporal changes of hydrochemistry during the three sampling campaigns were observed at SG10 ( Fig. 2 ), which is located in the groundwater depression. There were rising proportions of Cl with decreasing groundwater levels in August 2007, which may be related to upconing of deep salty groundwater or to saltwater intrusion from the northern part of the drawdown cone. By contrast, DG04 and SG12 ( Fig. 2 ), which are also located in the drawdown cone, do not show significant variations. This reflects the complex nature of subsurface conditions and is related to the spatial distribution of geological strata and changes in groundwater level caused by different intensities of groundwater exploitation through the year. 4.2 Cl/Br ratios and origin of salt There are several possible sources of salt in groundwater, such as seawater intrusion, mobilisation of formation water and evaporite dissolution from marine deposits. Chloride/Br − ratios may be used to determine the causes of groundwater salinisation for water with TDS higher than 2–3 g/L ( Cartwright et al., 2006; Alcalá and Custodio, 2008 ). The molar Cl/Br ratio of seawater is around 655 (Cl = 550 mmol/L, Br = 0.84 mmol/L, Siemann, 2003 ), which has been constant for all oceans since the Permian ( Drever, 1997 ) and can be taken as a reference value. Surface water and groundwater that have undergone evaporation show similar ratios until halite precipitates. Since Br − is less compatible in precipitating salts, it is enriched relative to Cl − in the residual brines, leading to Cl/Br ratios below 650. By contrast, precipitated salts are depleted in Br − , which causes Cl/Br ratios above 3000 for water that has dissolved halite ( Mazor, 1997 ). Molar Cl/Br ratios in all samples are most variable at low salinity (Cl < 46 mmol/L), ranging from 7 to 1132 ( Fig. 6 ). The lowest Cl/Br ratios in surface-water samples, up to 253 (SU02), were assumed to be the ratio of modern rainfall. Most fresh and brackish groundwater in the shallow aquifers has Cl/Br ratios lower than 253. The highest Cl/Br ratios, up to 1132, occur in groundwater with Cl − concentrations of <46 mmol/L ( Fig. 6 ). Dissolution of small volumes of halite from the unsaturated zone is the most likely mechanism that increases Cl/Br ratios. However, halite has molar Cl/Br ratios of ∼10 4 in many areas ( McCaffrey et al., 1987; Kloppmann et al., 2001; Cartwright et al., 2004 ); hence the amount of halite dissolution must be minor. Brines (584–2435 mmol/L) have Cl/Br ratios of 253–667 with a mean value of 503 that is generally lower than the oceanic ratio of ∼650, showing relative invariance with increasing Cl − concentrations. It confirms that evaporation is the dominant mechanism increasing salinity of the brine groundwater. Additionally, away from coastal areas, groundwater commonly has Cl/Br ratios lower than the ocean value. Groundwater with TDS below 2 g/L from shallow depths has a large range of Cl/Br ratios, mostly below seawater. This is probably related to anthropogenic input of Br − from agriculture indicated by elevated NO 3 - concentrations (e.g., SG07 with NO 3 - 246 mg/L and SG30 with NO 3 - 228 mg/L). As discussed by Thomas (1994) Cl/Br ratios cannot be used to identify the salt origin in these samples. Almost all Cl/Br ratios of samples with TDS above 2 g/L plot close to seawater, suggesting that relict seawater or recent seawater are important sources of salt rather than evaporite dissolution ( Fig. 6 ). One sample of deep saline water (DG06) plots below seawater, probably as a result from mixing with shallow water contaminated with anthropogenic Br − . 4.3 2 H/ 18 O isotopes and recharge conditions Groundwater has δ 18 O values of −9.7‰ to −1.3‰ and δ 2 H values of −72.7‰ to −21.0‰ ( Table 1 , Fig. 7 ). The local meteoric water line (LMWL, δ 2 H = 7.8 δ 18 O + 6.3, n = 8, r 2 = 0.78 from March to October) is based on δ 18 O and δ 2 H values of the average monthly rainfall monitored between 1986 and 1990 at Yantai station (37°32′24″N, 121° 24′00″E) some 250 km NE of Changyi city (data from International Atomic Energy Agency/World Meteorological Organisation (IAEA/WMO, 2006) . Yantai station has similar climatic and coastal conditions. Almost all samples plot on a line below the LMWL between end-members represented by low-mineralised freshwater (SG04), seawater and brines ( Fig. 7 ). Fresh groundwater is depleted in both 18 O and 2 H, and there is an increasing trend in both δ 18 O and δ 2 H in groundwater samples with increasing salinity from fresh water to brine water. This reflects the increasing influence of evaporation as a major process producing salinity. Seawater represents an end-member enriched in isotopes and plots far below the LMWL. The strong evaporitic fingerprint of seawater is due to the fact that the samples were taken at a very shallow depth below 1 m very close to the shoreline. The group of deep saline water samples (DG01, DG02, DG03, DG05, DG06), which are all located north of the depression, shows depleted isotopic signatures compared to modern seawater (δ 18 O ranging from −8.9‰ to −4.3‰, δ 2 H ranging from −67.8‰ to 62.8‰). On the other hand, these samples have Cl/Br ratios similar to seawater. Here the depleted isotopic signature is assigned to dilution of the palaeo-ocean water which may occur along continental margins by continental runoff. For example, Dakin et al. (1983) reported δ 18 O values of seawater about −10.5‰ in Georgia Strait between Vancouver Island and the Mainland. Therefore, the deep saline groundwater is presumed to have originated by seawater intrusion during the last sea level rise after the last glacial period around 10–15 ka ago ( Yechieli et al., 2009 ). Earlier infiltration of seawater during the previous interglacial periods with higher seawater level is not supported by the 14 C data discussed below. The brine samples plot on a line between seawater and freshwater in spite of showing a higher salinity than seawater. Chloride/Br ratios close to seawater ratios indicate that elevated TDS contents result from evapoconcentration. Therefore, the question is how to explain the depleted isotope signatures, assuming that evapoconcentration increases both salinity and isotope enrichment. Also in this case, a depleted initial seawater reservoir due to mixing with depleted runoff from the continental margin in colder periods may account for the combination of low δ 18 O values and high salinities of the brines. Therefore, the modern brines are assumed to have also evolved from evapoconcentration and subsequent intrusion of a palaeo-seawater reservoir after the formation of deep saline water discussed above. Fig. 7 also shows that river water samples are characterised by similar isotopic signatures to those of shallow groundwater. Results suggest that groundwater is recharged to some extent by river water, although the more elevated TDS of fresh groundwater also indicates mixing with more highly mineralised groundwater. 4.4 Origin of sulphate and δ 34 S variations Possible sources of SO 4 2 - in groundwater in Laizhou Bay are evaporite dissolution, sulfide oxidation or mixing with seawater and brines. Furthermore, the SO 4 2 - concentrations can be reduced by SO 4 2 - reduction. Numerous case studies have been conducted related to isotopic composition of SO 4 2 - in groundwater ( Müller et al., 1966; Fontes and Zuppi, 1976; Hendry et al., 1989; Fennell and Bentley, 1998 ) with the objective of determining sources of SO 4 and redox processes. Sulphate minerals (gypsum, anhydrite, etc.) typically have δ 34 S SO4 values between +9‰ and +24‰ ( Shi et al., 2004 ). Sulphate of modern marine origin should have δ 34 S SO4 values close to 21‰, the value of current ocean water SO 4 ( Rees et al., 1978 ), whereas older sulphates of marine origin such as Permian sulphates can have quite different signatures. In general, δ 34 S SO4 values of recycled evaporates range from +8 to +30‰ ( Mayer, 2005 ) with an average value of +13.4‰ ( Taberner et al., 2000 ). Sulphate reduction leads to enrichment in δ 34 S SO4, whereas sulfide oxidation results in negative δ 34 S SO4 values ( Clark and Fritz, 1997 ). To identify the SO 4 origin and chemical redox reactions in the groundwater, 26 water samples were selected for δ 34 S analysis, including eight fresh, 13 brackish, one seawater and three saline water samples ( Table 5 ). Except for DG04 and SG29, most δ 34 S data range between 10‰ and 21‰ ( Fig. 8 ). Deep saline water plots in a narrow range slightly below 21‰, supporting a seawater origin of salinity consistent with the other parameters discussed above. Also the brine sample plots very close to the seawater composition, which favours a relict seawater origin of the SO 4 that concurs with the other findings. The slight depletion in δ 34 S compared to modern seawater can be attributed to pyrite oxidation, which agrees with field data reporting pyrite in sediment samples and suboxic conditions. Since the coastal plain was much wider during recent lower seawater levels, pyrite is likely to have originated in reducing environments. Furthermore, saline and brine water has ORP values ranging from 9 to 120 mV, indicating weak oxidising conditions ( Fig. 9 ). The depleted signature of DG 04 (δ 34 S SO4 values near 0‰), located near a major print works, is probably related to anthropogenic SO 4 , such as SO 4 additives from water supply treatment, or soap and washing powder in domestic waste water. In contrast to saline and brine water, shallow fresh and brackish groundwater has a broad range of δ 34 S SO4 values between 10‰ and 17‰. Since the site-specific continental SO 4 signature is unknown, several processes can be assumed to produce this variety. Figs. 8 and 10 show that the δ 34 S SO4 increases with increasing mineralisation and decreasing SO 4 2 - /Cl − ratios, indicating that mixing processes of freshwater (low δ 34 S SO4 , high SO 4 2 - /Cl − ) with brines (high δ 34 S SO4 , low SO 4 2 - /Cl − ratio) are mostly responsible for S isotope variations of these samples. However, intermediate values about 10‰ could also be due to a mixture of pyrite-oxidation-derived SO 4 and marine SO 4 , which concurs with ORP data compiled in Fig. 9 . The very depleted signature of SG29 (depth 50 m) with a δ 34 S SO4 value of −7.19‰ is attributed to sulfide oxidation and/or anthropogenic activity. Enrichment of δ 34 S SO4 due to SO 4 reduction is not reflected in the data. In conclusion, although δ 34 S SO4 insufficiently distinguishes between continental and marginal SO 4 , Cl/Br ratios point to seawater as a sulphate source. 4.5 Tritium and 14 C ages of groundwater Tritium ( t 1/2 12.43 a) has been widely used to estimate young groundwater residence time ( Zuber, 1986; Szabo et al., 1996; Plummer et al., 1998 ). The presence of 3 H in groundwater suggests either younger ages than 50 a or mixing with young water. The 3 H data for precipitation from two GNIP stations in the study area showing similar climatic conditions was used. Analyses were available from 1986 to 1991 at Yantai Station and from 1985 to 2002 at Shijiazhuang Station showing a mean content 3 H of 16 TU in 2002, which is close to the background value for the atmosphere ( Jia et al., 1989; IAEA/WMO, 2006 ). Thirty-eight water samples have been analysed for 3 H contents, and are documented in Table 2 . The 3 H content of deep groundwater (0.7–3.5 TU) is lower than that of shallow groundwater (1.8–15.3 TU) and surface water (1.7–7.8 TU). The TU values of saline water from deep aquifers are mostly lower than 1 TU. Fig. 11 shows considerable contents of 3 H (∼15 TU) in shallow samples (depth <70 m), whereas deep water samples have 3 H contents below 3 TU or are 3 H-free. Furthermore, a general negative correlation of 3 H with depth and Cl concentration can be observed. These findings indicate that deep groundwater is isolated from modern recharge and has a residence time of more than 50 a. The wide range of 3 H contents in shallow fresh- and brackish water is mainly due to mixing of water of different ages. Shallow brackish water may have originated from mixing of old brines with young fresh groundwater in different proportions. The brine waters with 2.3–3.5 TU indicate mixing of old brines with young groundwater. Beside natural mixing processes, this could also be due to either contamination by water of domestic origin, hydraulic coupling by boreholes or mixing with 3 H-rich groundwaters that have been drawn into the sampled zone by pumping. Most shallow and dilute groundwaters (i.e., low-Cl concentration) in recharge areas contain 5.9–15.3 TU (mean 8.5 TU) ( Fig. 11 b). Carbon isotopic data were determined for 20 groundwater samples ( Table 2 ). Most shallow water samples with 3 H > 3 TU have a 14 C activity higher than 70 pmC ( Fig. 12 ). These waters are probably modern (<50 a) or contain a significant percentage of water recharged after the period of nuclear bomb tests. The 14 C ages of these samples are also given in Table 3 for reference purposes. The contribution of “dead” C from the aquifer matrix was constrained using δ 13 C values. For the North China Plain, values of soil gas δ 13 C are between −17‰ and −21‰ ( Chen et al., 2003 ), and the mean value of −19‰ was adopted. The δ 13 C of the carbonate end-member was taken as 1.5‰ ( Chen et al., 2003 ). Table 3 compares 14 C ages calculated by five different methods. Shallow groundwater turns out to be of modern age according to the methods of Tamers (1975) , CMB (chemical mass-balance correction; Fontes and Garnier, 1979 ) and Pearson and Hanshaw (1970) , whereas the STAT (statistical correction; Vogel, 1970 ) approach and uncorrected ages give much older ages not supported by 3 H data above 2 TU ( Table 3 ). With regard to deep groundwater, the ages corrected after Pearson and Hanshaw (1970) yielded age values in samples with very low 14 C concentrations, indicating old, isolated groundwater (DG04, DG05, DG08 and DG09). The calculated ages of these samples concur with results obtained by the other methods. It is assumed that elevated 14 C values are related to mixing with younger water, which is also supported by the 3 H data of these samples. The Tamers (1975) model assumes calcite dissolution under closed conditions. It gives the most reliable results for deep groundwater samples and is in agreement with the results of the STAT approach. The different age correction methods yielded broadly similar ages for most of the groundwater samples. Hence, a ‘mean age’ is calculated for each sample in Table 3 . The ages of two brine samples (SG41 and DG07) are 2.3 ka and 7.0 ka, respectively. The 14 C age would be from 7.5 ka to 15.2 ka for saline water and from modern age to 21.7 ka for brackish water. The variations in corrected 14 C ages with depth and distance from the coastline are shown in Fig. 13 (data from the mean age values in Table 3 ). Most fresh groundwater falls in the modern groundwater range along the groundwater flow path, indicating fast circulation. Brackish groundwater in the shallow aquifer shows modern ages (modern to ∼3.4 ka) in groundwater close to the drawdown cone and about 16.6–20.3 ka between the drawdown and the ocean. The deep saline groundwater (80–125 m depth) has ages of about 7.5–15.2 ka, and the age of brine water is more than 2.3 ka. There is a vertically increasing trend in the brine water ages (from SG41 at 30 m depth to DG07 at 60 m depth), indicating that the stable thickness of the clay layer strongly limits vertical mixing. 4.6 Mixing In the δ 18 O vs. Cl plot ( Fig. 14 ) four different end-members are visible: (i) freshwater, (ii) brines, (iii) deep saline water and (iv) seawater. Brackish groundwater is plotted between these end-members. Based on the analysed hydrochemical composition, three mixing scenarios are proposed to explain the distribution of the samples ( Fig. 15 a). Mix 1: Figs. 14 and 15 a show that a large part of brackish water in the drawdown cone (SG36, SG39, SG48; SG17, SG19) lies on a mixing line between freshwater and deep saline water and is not influenced by intrusion of modern seawater or brines. This indicates that upconing deep saline water has a greater effect on salinisation than shallow brine water. The δ 18 O variability of the freshwater end-members is reflected also in the δ 18 O variability of brackish water samples mixed with deep saline water. Only one sample located in the groundwater depression (SG38) indicates mixing with modern seawater. Mix 2: Saline water samples SG38, DG05 and Z3-3 (20 m depth) lie on a freshwater–seawater mixing line ( Fig. 15 a). DG05 is located between the depression and the sea at 125 m depth, whereas SG 38 is from the depression, indicating preferential intrusion of seawater into areas of major groundwater extraction. Note that DG05 shows mixing with deep saline water in July 2006 but mixing with modern seawater in July 2007. Mix 3: Saline water between the drawdown cone and the coastline shows different origins of salt. Z3-1 (60–85 m depth) and SG49 plot on a mixing line between freshwater and deep brine water. SG 49 is located very close to the Wei River, which presumably constitutes the discharge area for deep brine water. Provided that the Cl − ion concentration remains unaffected by evaporite dissolution or anthropogenic inputs, this parameter can be regarded, in addition to δ 18 O, as a reference parameter for estimating the contribution of the different sources. Nonetheless, δ 18 O shows a high variability in freshwater which significantly limits mixing calculations for individual samples without detailed knowledge of local flow paths, which is beyond the scope of this study. 4.7 Conceptual model The results of this study provide a basis for a unifying conceptual model that describes the major hydrochemical and physical processes along a cross-section from north to south parallel to the principal flow system ( Fig. 16 ). The fact that the regional salinity distribution is in broad agreement with the general flow pattern indicates that three-dimensional flow is only of local importance and does not affect the overall findings. The compositions of groundwater from the coastal aquifers ( Fig. 16 a) represent the principal part of the data set used in this work. The distribution of isotopic composition for various water bodies is illustrated schematically in Fig. 16 b. In the southern part of the shallow aquifer, low-Cl- and 3 H-bearing groundwaters represent the isotopic composition of modern precipitation (δ 18 O = −11‰ to −12‰), indicating that these groundwaters were recharged during warm climatic periods such as the present. Fig. 16 b illustrates the main mixing processes associated with the two drawdown cones determining the salt distribution in the aquifer system. The increase in salinity, Cl/Br ratios and isotope ratios of 2 H/ 18 O in brines point to relict seawater as an important source of salt. On the other hand, it is obvious that the shallow brine water also mixes with meteoric water with 14 C showing 62.7 pmC and 3 H above 1 TU. The spatial pattern of groundwater flow becomes complex towards the coastline due to regional confining units composed of fine-grained silts and clay layers with increased thickness. Therefore, north of the drawdown cone, confining units lead to a vertical separation of the hydrochemistry. The shallow groundwater composition indicates mixing between freshwater and modern seawater, whereas in the deeper part brackish water results from mixing between deep saline water and freshwater. Due to the presence of brines, salinisation of the aquifer would also occur under natural conditions without groundwater extraction; however, results of this and previous studies show that salinisation migrates inland mainly as a result of ascending deep saline water. 4.8 Inverse hydrogeochemical modelling of three mixing scenarios Inverse modelling simulations were performed with PHREEQC-2 ( Parkhurst and Appelo, 1999 ). In inverse modelling, one initial aqueous solution is assumed to mix with other initial aqueous solutions and to react with minerals and gases to produce the observed composition of a final aqueous solution. Inverse modelling calculates mixing fractions for the aqueous solutions and mole transfers of the gases and minerals that produce the composition of the final aqueous solution. The basic approach in inverse modelling is to account for the changes in moles between initial and final solutions by the dissolution or precipitation of minerals ( Garrels and Mackenzie, 1967; Parkhurst et al., 1982 ). As a result, PHREEQC-2 may deliver zero, one or more possible models with mole transfers explaining the difference between initial and final aqueous solutions. PHREEQC-2 does not consider saturation indices, and, therefore, model results should always be checked for their thermodynamical feasibility. The mixing scenarios proposed in Section 4.6 are based on two conservative parameters, Cl and δ 18 O, but do not account for possible chemical reactions and changing concentrations of other parameters involved in these reactions. Therefore, inverse modelling was carried out to identify hydrochemical reactions that account for changes in water chemistry taking place during mixing and interaction of water with the present mineral assemblage of the aquifer. Furthermore, the thermodynamic feasibility of the mixing scenarios proposed in Section 4.6 was verified, considering also the reactive components of the water samples involved. To perform the simulations, PHREEQC requires the definition of one or more initial aqueous solutions, one final aqueous solution and a set of minerals and/or gases that may be present. Here, the initial aqueous solutions represent the end-members whose mixture, combined with chemical reactions, accounts for the hydrochemical composition of the final aqueous solution. The water samples used to define initial and final solutions for the inverse scenarios are compiled in Table 6 . The model input files consist of field-measured pH, temperature and major ion concentrations of each input solution. The number of models obtained with each modelling exercise run with PHREEQC-2 is also shown in Table 6 . The criterion followed to select the best model was basically to choose the thermodynamically feasible model with the smallest number of mineral phases and exchangers. In all scenarios dolomite, calcite and gypsum were defined as representative minerals of the aquifer material at the study site. The possibility of dissolution or precipitation of the respective phases was defined according to the calculated saturation indices of the input solutions. Calcite was oversaturated in the input solutions of all simulations, whereas gypsum and dolomite were undersaturated. Therefore, calcite was only allowed to precipitate (but not to dissolve), whereas gypsum and dolomite were only allowed to dissolve ( Table 7 ). Sinks and sources of inorganic C are considered by defining the CO 2 phase. Furthermore, all simulations included the possibility of cation exchange between Ca, Mg and Na related to saltwater intrusion or refreshening aquifer conditions as discussed above. To test the presented hypothetical conceptual model and to assess the hydrochemical reactions to the three mixing scenarios documented in Fig. 15 a, the following scenarios were calculated with PHREEQC-2 including Cation Exchange ( Table 6 ). The PITZER database can be used to account for highly mineralised aqueous solutions with a ionic strength above 0.5. 4.8.1 Scenario 1 (Mix 1 in Fig. 15 a): Evolution of shallow brackish water from mixing of freshwater with deep saline water As indicated in Fig. 15 b and discussed in the conceptual model, the chemical evolution observed in shallow brackish water is assumed to be a result of mixing between freshwater and deep saline water. To verify this hypothesis and to identify possible chemical reactions that occur during the mixing process, scenario 1 was set up. Shallow brackish water located in the Changyi depression (sample SG36) was defined as the final solution resulting from the mixing between the two initial end-member solutions freshwater (sample SG40) and deep saline water north of the depression cone (DG06). The possibility of cation exchange between Ca, Mg and Na related to saltwater intrusion or refreshening aquifer conditions was included in the simulation. In this scenario a thermodynamically feasible solution was found when the mineral phases and ion exchange were introduced as indicated in Tables 6 and 7 . The simulations suggest mixing proportions of 0.8735 (SG40) and 0.1266 (DG06), dissolution of dolomite and gypsum and ion exchange of Ca and Mg for Na. This reflects intrusion of freshwater into a saline aquifer leading to Na release into intruding freshwater. 4.8.2 Scenario 2 (Mix 2 in Fig. 15 a): Evolution of saline water from mixing of freshwater and seawater The second inverse scenario tries to reproduce saline water sample SG 38 sampled in the Changyi depression and located on the mixing line between fresh- and seawater. In this scenario, three thermodynamically feasible solutions were found when the mineral phases and ion exchange were introduced as indicated in Table 6 . The simulations suggest mixing proportions of 0.49 (SG40) and 0.51 (SW) combined with dissolution of dolomite and gypsum to reproduce saline water (SG 38). Ion exchange of Ca for Na points to intrusion of freshwater into a saline aquifer. 4.8.3 Scenario 3 (Mix 3 in Fig. 15 a): Evolution of shallow saline water from mixing of freshwater and deep brine water The third inverse scenario was set up to verify the hypothesis that the formation of shallow saline water represented by SG49 results from mixing freshwater (SU09) with deep brines (501-6). Like scenario 2, results indicate mixing proportions of 0.67 (SU09) and 0.33 (501-6), dissolution of dolomite and gypsum and ion exchange of Ca for Na. Modelling results show that all hypothetical mixing scenarios derived from conservative components were verified by the simulations. In all scenarios, mixing, dissolution of dolomite and gypsum and calcite precipitation account for the changes in moles between initial and final aqueous solutions. 5 Conclusions The present study uses hydrochemical and isotopic evidence to explore groundwater salinisation processes in the Quaternary aquifer on the south coast of Laizhou Bay. The study describes a complex coastal aquifer system showing a variety of groundwater compositions with different ages, isotopic signatures and salinities. The general flow path towards the coast is disrupted by two groundwater depressions due to groundwater exploitation leading to intrusion of deep saline water, seawater and brines into the shallow freshwater aquifer system. The origin of salinity is complex and could only be assessed by the simultaneous evaluation of several hydrochemical and isotopic parameters. The combination of δ 18 O and Cl data enabled four different end-members to be identified: freshwater, brines, deep saline water, seawater and their respective mixtures. Chloride/Br ratios close to modern seawater were applied to identify the evapoconcentration of former seawater as the main process producing salinity in groundwater. Nonetheless, brines and especially deep saline water show very depleted isotopic ratios compared to modern seawater, indicating that intrusion of modern seawater would not account for this isotopic depletion. Therefore, an isotopically depleted, initial seawater reservoir due to continental runoff in colder periods, subsequent evapoconcentration and palaeo-seawater intrusion is assumed to have produced the salinity in present brines and deep saline groundwater. Carbon-14 data of deep, 3 H-free, saline groundwater yield ages of about 15 ka, pointing to seawater intrusion having occurred during sea level rise since the last glacial period around 10–15 ka ago. By contrast, brine water shows a wide range of 3 H contents and 14 C ages depending on the sampling depth. This range is mainly due to the complex conditions of mixing, and no strict isolation from modern groundwater was observed. Although the low isotopic signatures in the brines compared to modern seawater also require an initially depleted seawater reservoir, 14 C dating and higher salinities reveal a later event of seawater intrusion characterised by a higher degree of evapoconcentration. The δ 18 O and Cl data indicate that most salinisation of the brackish groundwater in the Changyi drawdown cone stems from mixing of freshwater with ascending deep saline water of older age. However, mixing with seawater was observed in one sample of the groundwater depression, pointing to preferential flow of intruding seawater. Hydrochemical and stable isotope data of samples taken between the drawdown cone and the coast point to formation of saline water due to mixing of freshwater with brines and seawater. One sample showed a temporal change of mixing with seawater and brines from 2006 to 2007. Results suggest that seawater and brines coexist in this part of the study area, indicating preferential pathways of the different water types. The δ 34 S SO4 data support seawater intrusion as a possible source of SO 4 2 - , although this method cannot exclude mineral dissolution. Mixing scenarios were verified by hydrochemical simulations quantifying processes of mixing, dolomite/gypsum dissolution, calcite precipitation and ion exchange to account for the changes in moles between initial and final aqueous solutions. The present research shows how the application of combined parameters improves the identification of (i) salt sources, (ii) individual end-members and their hydrochemical evolution, (iii) different events of palaeo-seawater intrusion, and (iv) mixing proportions between these end-members. It further demonstrates the temporal change of isotopic compositions in seawater with limited connection to the ocean and its effect on the groundwater composition after intrusion. A refined understanding of the dynamics in such complex hydrogeological systems is essential to assess the impact of anthropogenic measures on salinisation. The methodology used here can also be applied to other field sites and may help to provide a reasonable basis for effective control measures and sustainable water management. Acknowledgements This study was financially supported by the National Natural Science Foundation of China (40801018), and National ‘973 Program’ (No. 2010CB428805), and by the project of the China Geology Survey entitled “Assessment of Vulnerability and Investigation of Environmental Geology in the Key Section of Circum-Bohai-Sea Region”. The authors would like to thank Ian Cartwright, Chen Zongyu, Matthew J. Currell, and two anonymous reviewers for their helpful comments and review of the manuscript. The authors are grateful to Dr. Wang Peng, Dr. Liu Xin, and Dr. Xie Hailan, and Pan Tong, Tianjin Institute of Geology and Mineral Resources, for their help and support during water sampling in the field and data collection. Appendix A Supplementary material Supplementary data associated with this article can be found, in the online version, at doi:10.1016/j.apgeochem.2011.02.007 . Appendix A Supplementary material Supplementary Table 1 Basic information of groundwater sampling network. Supplementary Table 2 Main characteristics and chemical composition of sampled water. References Alcalá and Custodio, 2008 F.J. Alcalá E. Custodio Using the Cl/Br ratio as a tracer to identify the origin of salinity in aquifers in Spain and Portugal J. Hydrol. 359 2008 189 207 Appelo and Postma, 2005 C.A.J. Appelo D. Postma Geochemistry, Groundwater and Pollution second ed. 2005 Taylor & Francis Group Bergelson et al., 1999 G. Bergelson R. Nativ A. Bein Salinization and dilution history of groundwater discharging into the Sea of Galilee, the Dead Sea Transform, Israel Appl. Geochem. 14 1999 91 118 Cartwright et al., 2006 I. Cartwright T.R. Weaver L.K. Fifield Cl/Br ratios and environmental isotopes as indicators of recharge variability and groundwater flow: an example from the southeast Murray Basin, Australia Chem. Geol. 231 2006 38 56 Cartwright et al., 2004 I. Cartwright T.R. Weaver S. Fulton C. Nichol M. Reid X. Cheng Hydrogeochemical and isotopic constraints on the origins of dryland salinity, Murray Basin, Victoria, Australia Appl. Geochem. 19 2004 1233 1254 Chen et al., 1997 H.H. Chen Y.X. Zhang X.M. Wang Z.Y. Ren L. Li Salt-water intrusion in the lower reaches of the Weilhe River, Shandong Province, China Hydrogeol. J. 5 3 1997 82 88 Chen et al., 2003 Z.Y. Chen J.X. Qi J.M. Xu J.M. Xu H. Ye Y.J. Nan Paleoclimatic interpretation of the past 30 ka from isotopic studies of the deep confined aquifer of the North China Plain Appl. Geochem. 18 2003 997 1009 Clark and Fritz, 1997 I.D. Clark P. Fritz Environmental Isotopes in Hydrogeology 1997 Lewis Publishers Boca Raton pp. 144–207 Coplen, 1988 T.B. Coplen Normalization of oxygen and hydrogen isotope data Chem. Geol. 72 1988 293 297 Craig, 1961 H. Craig Standard for reporting concentration of deuterium and oxygen-18 in natural water Science 133 1961 1833 1834 Currell et al., 2010 M.J. Currell I. Cartwright D.C. Bradley D.M. Han Recharge history and controls on groundwater quality in the Yuncheng Basin, north China J. Hydrol. 385 2010 216 229 Custodio and Bruggeman, 1987 Custodio, E., Bruggeman, G.A., 1987. Groundwater problems in coastal areas. In: Studies and Reports in Hydrology (UNESCO), No.45, International Hydrological Programme (IHP), Paries. Dakin et al., 1983 R.A. Dakin R.N. Farvolden J.A. Cherry P. Fritz Origin of dissolved solids in groundwater of Mayne Island, British Columbia, Canada J. Hydrol. 63 1983 233 270 Drever, 1997 J.I. Drever The Geochemistry of Natural Waters: Surface and Groundwater Environments 1997 Prentice-Hall New Jersey, USA Edmunds and Smedley, 2000 W.M. Edmunds P.L. Smedley Residence time indicators in groundwater; the East Midlands Triassic sandstone aquifer Appl. Geochem. 15 2000 737 752 Essaid, 1990 H.I. Essaid A multilayered sharp interface model of coupled freshwater and saltwater flow in coastal systems: model development and application Water Resour. Res. 26 1990 1431 1454 Fennell and Bentley, 1998 J. Fennell L.R. Bentley Distribution of sulfate and organic carbon in a prairie till setting: natural versus industrial sources Water Resour. Res. 34 1998 1781 1794 Fetter, 1994 Fetter, C.W., 1994. Applied Hydrogeology. Englewood Cliffs, Prentice Hall. Fontes and Garnier, 1979 J.C. Fontes J.M. Garnier Determination of the initial 14C activity of total dissolved carbon: a review of existing models and a new approach Water Resour. Res. 15 1979 399 413 Fontes and Zuppi, 1976 Fontes, J.C., Zuppi, G.M., 1976. Isotopes and Water Chemistry in Sulphide-Bearing Springs of Central Italy. Interpretation of Environmental Isotope and Hydrochemical Data in Ground Water Hydrology, Proc. Advisory Group Meeting. IAEA, Vienna, Austria, pp. 143–158. Garrels and Mackenzie, 1967 Garrels, R.M., Mackenzie, F.T., 1967. Origin of the chemical compositions of some springs and lakes. In: Gould, R.F. (Ed.), Advances in Chemistry Series, No. 67, American Chemical Society, Washington, DC, pp. 222–242. Gates et al., 2008 J.B. Gates W.M. Edmunds W.G. Darling J. Ma Z. Pang A.A. Young Conceptual model of recharge to southeastern Badain Jaran Desert groundwater and lakes from environmental tracers Appl. Geochem. 23 2008 3519 3534 Grassi and Cortecci, 2005 S. Grassi G. Cortecci Hydrogeology and geochemistry of the multilayered confined aquifer of the Pisa plain (Tuscany – Central Italy) Appl. Geochem. 20 2005 41 54 Grassi et al., 2007 S. Grassi G. Cortecci P. Squarci Groundwater resource degradation in coastal plains: the example of the Cecina area (Tuscany – Central Italy) Appl. Geochem. 22 2007 2273 2289 Halas and Szaran, 1999 S. Halas J. Szaran Low-temperature thermal decomposition of sulfates to SO 2 for on-line 34 S/ 32 S analysis Anal. Chem. 71 1999 3254 3257 Han et al., 2001 F. Han Y.Q. Xue J.C. Wu Hydrochemical characteristics of groundwater in salt-water intrusion condition and genesis of brine along the south coast of the Laizhou Bay, China Geol. Rev. 47 1 2001 102 108 (in Chinese) Han et al., 1996 Y.S. Han G.L. Meng S.Q. Wang Quaternary Underground Brine in the Coastal Areas of the Northern China 1996 Science Press Beijing pp. 36–54 (Chapter 4, in Chinese) Hendry et al., 1989 M.J. Hendry H.R. Krouse M.A. Shakur Interpretation of oxygen and sulphur isotopes from dissolved sulfates in tills of Southern Alberta, Canada Water Resour. Res. 25 1989 567 572 IAEA/WMO, 2006 IAEA/WMO, 2006. Global Network of Isotopes in Precipitation. The GNIP Database, Vienna. < http://www-naweb.iaea.org/napc/ih/IHS_resources_gnip.html> . Ji, 1991 M.C. Ji Some changes of resources and environment caused by seawater intrusion Environ. Sci. 12 5 1991 62 66 (in Chinese) Jia et al., 1989 Y.K. Jia H.P. Zhang D.S. Wang E.K. Liu J.C. Sun Tritium distribution of precipitation in China Site Invest. Sci. Technol. 4 1989 9 12 Jones et al., 1999 B.F. Jones A. Vengosh E. Rosenthal Y. Yechieli Geochemical investigations J.A. Bear H.-D. Cheng S. Sorek D. Ouazar I. Herrera Seawater Intrusion in Coastal Aquifers – Concepts, Methods and Practices 1999 Kluwer 1 60 (Chapter 3) Kim et al., 2003 Y. Kim K.-S. Lee D.-C. Koh D.-H. Lee S.-G. Lee W.-B. Park G.-W. Koh N.-C. Woo Hydrogeochemical and isotopic evidence of groundwater salinization in a coastal aquifer: a case study in Jeju volcanic island, Korea J. Hydrol. 270 2003 282 294 Kloppmann et al., 2001 W. Kloppmann Ph. Négrel J. Casanova H. Klinge K. Schelkes C. Guerrot Halite dissolution derived brines in the vicinity of a Permian salt dome (N German Basin). Evidence from boron, strontium, oxygen, and hydrogen isotopes Geochim. Cosmochim. Acta 65 2001 4087 4101 Li et al., 2000 D.G. Li M.H. Zhao M. Han A.X. Jiang Z.L. Zhang A study of the shallowly-buried paleochannel zones in the south coast plain of the Laizhou Bay Marine Geol. Quatern. Geol. 20 1 2000 23 29 (in Chinese with English abstract) Ma et al., 2007 F.S. Ma Y.S. Yang R. Yuan Z. Cai S. Pan Study of shallow groundwater quality evolution under saline intrusion with environmental isotopes and geochemistry Environ. Geol. 51 2007 1009 1017 Marimuthu et al., 2005 S. Marimuthu D.A. Reynolds L.C. Le Gal A field study of hydraulic, geochemical and stable isotope relationships in a coastal wetlands system J. Hydrol. 315 2005 93 116 Mayer, 2005 B. Mayer Assessing source and transformations of sulfate and nitrate in the hydrosphere using isotope techniques P.K. Aggarwal J.R. Gat K.F.O. Froehlich Isotopes in the Water Cycle: Past, Present and Future of a Developing Science 2005 Springer Netherlands 7 89 (Chapter 6) Mazor, 1997 E. Mazor Chemical and Isotopic Ground-Water Hydrology 1997 Dekker NY McCaffrey et al., 1987 M.A. McCaffrey B. Lazar H.D. Holland The evaporation path of seawater and the coprecipitation of Br − and K + with halite J. Sed. Petrol. 57 1987 928 937 Meng et al., 1997 G.L. Meng Y.S. Han S.Q. Wang Types and district divisions of seawater intrusion concerning southern coast of Laizhou Bay J. Oceanog. Hunanhai Bohai Seas 15 2 1997 25 32 Möller et al., 2007 P. Möller E. Rosenthal S. Geyer A. Flexer Chemical evolution of saline waters in the Jordan-Dead sea transform and in adjoining areas Int. J. Earth Sci. (Geol. Rundsch.) 96 2007 541 566 Müller et al., 1966 G. Müller H. Nielsen W. Ricke Sulphur isotope abundance in formation waters and evaporates from northern and southern Germany Chem. Geol. 1 1966 211 220 Ning, 2004 J.S. Ning The Research of Chemical Characters of the Underground Brine along Laizhou Bay 2004 Masters Ocean Univ. China pp. 37–38 (in Chinese) Nonner, 2006 J.C. Nonner Introduction to Hydrogeology 2006 Taylor & Francis Group London, UK Parkhurst and Appelo, 1999 Parkhurst, D.L., Appelo, C.A.J., 1999. User’s Guide to PHREEQC – A Computer Program for Speciation, Reaction-Path, 1D-Transport, and Inverse Geochemical Calculation. US Geol. Surv. Water-Resour. Invest. Rep. 99-4259. Parkhurst et al., 1982 Parkhurst, D.L., Plummer, L.N., Thorstenson, D.C., 1982. BALANCE – A Computer Program for Calculating Mass Transfer for Geochemical Reactions in Ground Water. US Geol. Surv. Water-Resour. Invest. Rep. 82-14. Pearson and Hanshaw, 1970 Pearson, F.J., Hanshaw, B.B., 1970. Sources of dissolved carbonate species in groundwater and their effects on carbon-14 dating. In: IAEA (Ed.), Isotope Hydrology, IAEA, Vienna, pp. 271–285. Pinder, 1973 G.F. Pinder A Galerkin-finite element simulation of groundwater concentration on Long Island Water Resour. Res. 9 1973 1657 1669 Plummer et al., 1998 L.N. Plummer E. Busenberg S. Drenkard P. Scholosser B. Ekwurzel R. Weppirnig J.B. McConnell R.L. Michel Flow of river water into a karstic limestone aquifer. 2. Dating the young fraction in groundwater mixtures in the upper Floridan aquifer near Valdosta, Georgia Appl. Geochem. 8 1998 1017 1043 Pulido-Leboeuf, 2004 P. Pulido-Leboeuf Seawater intrusion and associated processes in a small coastal complex aquifer (Castell de Ferro, Spain) Appl. Geochem. 19 2004 1517 1527 Rees et al., 1978 C.E. Rees W.J. Jenkins J. Monster The sulphur isotopic composition of ocean water sulfate Geochim. Cosmochim. Acta 42 1978 377 381 Rozanski and Gröning, 2004 Rozanski, K., Gröning, M., 2004. Tritium assay in water samples using electrolytic enrichment and liquid scintillation spectrometry. In: IAEA-TECDOC-1401, Proc. Consultants Meeting on Quantifying Uncertainty in Nuclear Analytical Measurements, 11–14 May 1998, Vienna, IAEA, Vienna, pp. 195–217. Schiavo et al., 2009 M.A. Schiavo S. Hauser P.P. Povinec Stable isotopes of water as a tool to study groundwater-seawater interactions in coastal south-eastern Sicily J. Hydrol. 364 2009 40 49 Sherif and Hamza, 2001 M.M. Sherif K.I. Hamza Mitigation of seawater intrusion by pumping brackish water Transport Porous Media 43 2001 29 44 Shi et al., 2004 Z.S. Shi K.Y. Chen J. Shi H.J. He B.J. Liu Sulfur isotopic composition and its geological significance of the Paleogene sulfate rock deposited in Dongpu Depression Petrol. Explor. Develop. 31 6 2004 44 46 (in Chinese) Siemann, 2003 M.G. Siemann Extensive and rapid changes in seawater chemistry during the Phanerozoic: evidence from Br contents in basal halite Terra Nova 15 1 2003 243 248 Sivan et al., 2005 O. Sivan Y. Yechieli B. Herut B. Lazar Geochemical evolution and timescale of seawater intrusion into the coastal aquifer of Israel Geochim. Cosmochim. Acta 69 2005 579 592 Szabo et al., 1996 A. Szabo D.E. Rice L.N. Plummer E. Busenberg S. Drenkard P. Schlosser Age dating of ground water using chlorofluorocarbons, tritium/helium 3, and flow path analysis in an unconfined aquifer of the New Jersey coastal plain Water Resour. Res. 32 1996 1023 1038 Taberner et al., 2000 C. Taberner D.I. Cendón J.J. Pueyo C. Ayora The use of environmental markers to distinguish marine vs. continental deposition and to quantify the significance of recycling in evaporite basins Sed. Geol. 137 2000 213 240 Tamers, 1975 M.A. Tamers Validity of radiocarbon dates on groundwater Geophys. Survey 2 1975 217 239 Thomas, 1994 Thomas, L., 1994. Hydrogeochemische Untersuchungen an den Ölfeldwässern aus NW-Deutschland und dem Oberrheingraben und ihre Modellierung unter dem Aspekt der Entwicklung eines Expertensysthems für Fluid–Rock-Interactions (Diss.). Berliner Geowissenschaftliche Abhandlungen. Selbstverlag Fachbereich Geowissenschaften, Berlin. TJR, 2006 TJR, 2006. Report of “Study of Real-time Monitoring and Warning on Sea-Salt Water Intrusion in Laizhou Bay – A case study of Changyi-Liutuan Profile”, Tianjin Institute of Geology and Mineral Resources, pp. 37–38 (in Chinese) Vengosh et al., 2002 A. Vengosh J. Gill M. Lee Davisson G. Bryant Hudson A multi-isotope (B, Sr, O, H and C) and age dating ( 3 H– 3 He and 14 C) study of groundwater from Salinas Valley, California: hydrochemistry, dynamics, and contamination processes Water Resour. Res. 38 2002 9.1 9.17 Vogel, 1970 Vogel, J.C., 1970. Carbon-14 dating of ground water. In: Isotope Hydrology 1970, STI/PUB/255, IAEA, Vienna, Austria, pp. 225–239. Xue et al., 2000 Y.Q. Xue J.C. Wu S.J. Ye Y.X. Zhang Hydrogeological and hydrogeochemical studies for salt water intrusion on the South Coast of Laizhou Bay, China Ground Water 38 2000 38 45 Yechieli et al., 2009 Y. Yechieli U. Kafri O. Sivan The inter-relationship between coastal sub-aquifers and the Mediterranean Sea, deduced from radioactive isotopes analysis Hydrogeol. J. 17 2009 265 274 Zhang et al., 1996 Y.X. Zhang Y.Q. Xue H.H. Chen Deposit seawater characteristics in the Strata and its formation environment in the South Coastal Plain of Laizhou Bay since late Pleistocene Acta Oceanol. Sinica 18 6 1996 61 68 (in Chinese) Zhang and Peng, 1998 Z.L. Zhang L.M. Peng The groundwater hydrochemical characteristics on seawater intruded in eastern and southern coasts of Laizhou Bay China Environ. Sci. 18 2 1998 121 125 (in Chinese) Zhao, 1996 Zhao, D.S., 1996. Research on Disaster Protection for Seawater Intrusion. Shandong Press of Sciences and Technology, Jinan, pp. 3–4 (in Chinese). Zuber, 1986 Zuber, A., 1986. Mathematical models for the interpretation of environmental radioisotopes in groundwater systems. In: Fritz, P., Fontes, J.C. (Eds.), Handbook of Environmental Isotope Geochemistry, vol. 2. Elsevier Science Publishers, Amsterdam, pp. 1–59.
更多查看译文
关键词
spatial context,surface water,cross section,thermodynamics,conceptual model,residence time,ion exchange
AI 理解论文
溯源树
样例
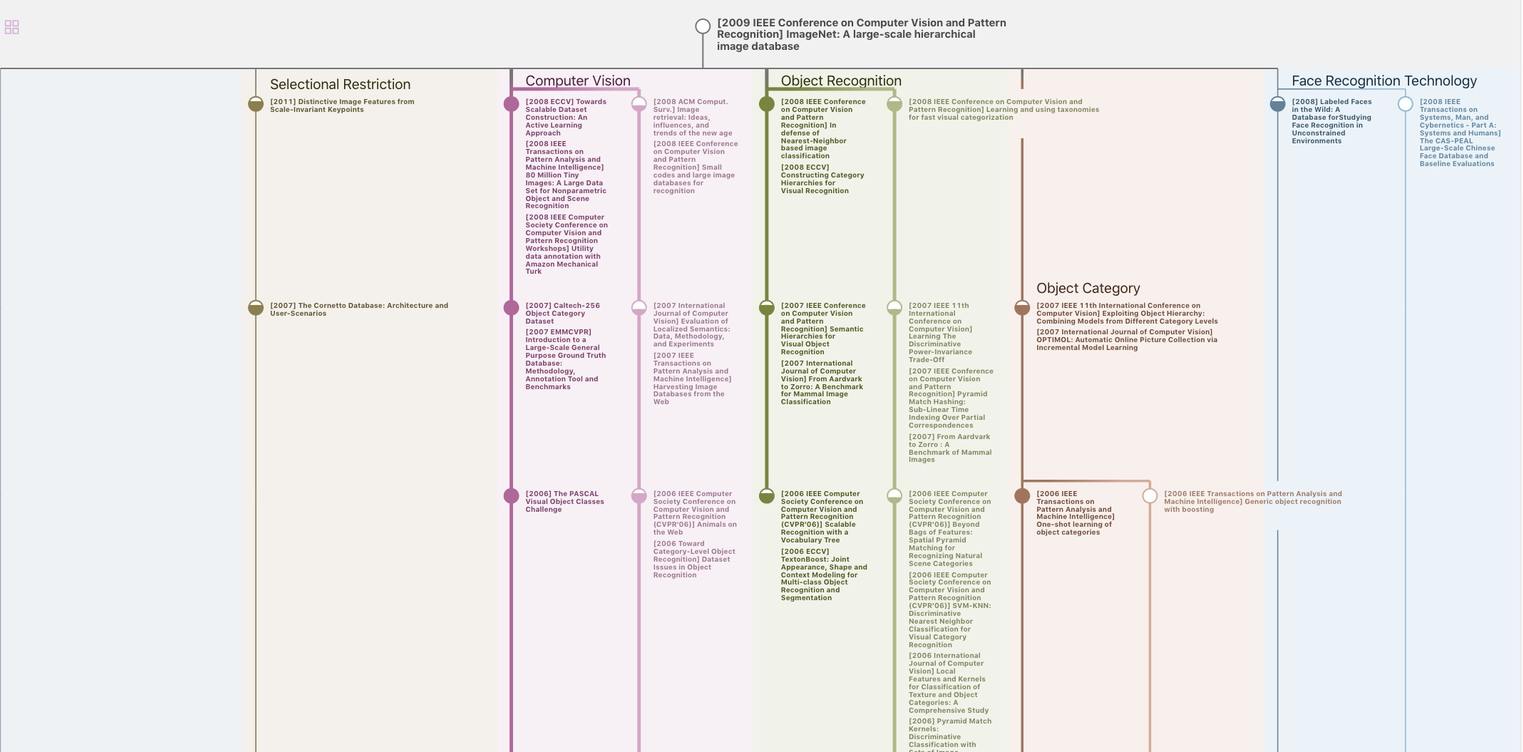
生成溯源树,研究论文发展脉络
Chat Paper
正在生成论文摘要