Author response: Single-cell analysis uncovers that metabolic reprogramming by ErbB2 signaling is essential for cardiomyocyte proliferation in the regenerating heart
openalex(2019)
摘要
Article Figures and data Abstract eLife digest Introduction Results Discussion Materials and methods Data availability References Decision letter Author response Article and author information Metrics Abstract While the heart regenerates poorly in mammals, efficient heart regeneration occurs in zebrafish. Studies in zebrafish have resulted in a model in which preexisting cardiomyocytes dedifferentiate and reinitiate proliferation to replace the lost myocardium. To identify which processes occur in proliferating cardiomyocytes we have used a single-cell RNA-sequencing approach. We uncovered that proliferating border zone cardiomyocytes have very distinct transcriptomes compared to the nonproliferating remote cardiomyocytes and that they resemble embryonic cardiomyocytes. Moreover, these cells have reduced expression of mitochondrial genes and reduced mitochondrial activity, while glycolysis gene expression and glucose uptake are increased, indicative for metabolic reprogramming. Furthermore, we find that the metabolic reprogramming of border zone cardiomyocytes is induced by Nrg1/ErbB2 signaling and is important for their proliferation. This mechanism is conserved in murine hearts in which cardiomyocyte proliferation is induced by activating ErbB2 signaling. Together these results demonstrate that glycolysis regulates cardiomyocyte proliferation during heart regeneration. eLife digest Heart attacks are a common cause of death in the Western world. During a heart attack, oxygen levels in the affected part of the heart decrease, which causes heart muscle cells to die. In humans the dead cells are replaced by a permanent scar that stabilizes the injury but does not completely heal it. As a result, individuals have a lower quality of life after a heart attack and are more likely to die from a subsequent attack. Unlike humans, zebrafish are able to regenerate their hearts after injury: heart muscle cells close to a wound divide to produce new cells that slowly replace the scar tissue and restore normal function to the area. It remains unclear, however, what stimulates the heart muscle cells of zebrafish to start dividing. To address this question, Honkoop, de Bakker et al. used a technique called single-cell sequencing to study heart muscle cells in wounded zebrafish hearts. The experiments identified a group of heart muscle cells close to the site of the wound that multiplied to repair the damage. This group of cells had altered their metabolism compared to other heart muscle cells so that they relied on a pathway called glycolysis to produce the energy and building blocks they needed to proliferate. Blocking glycolysis impaired the ability of the heart muscle cells to divide, indicating that this switch is necessary for the heart to regenerate. Further experiments showed that a signaling cascade, which includes the molecules Nrg1 and ErbB2, induces heart muscle cells in both zebrafish and mouse hearts to switch to glycolysis and undergo division. These findings indicate that activating glycolysis in heart muscle cells may help to stimulate the heart to regenerate after a heart attack or other injury. The next step following on from this work is to develop methods to activate glycolysis and promote cell division in injured hearts. Introduction Within the animal kingdom, regenerative capacity of damaged organs and body parts differs widely. While regenerative capacity is generally low in mammalian species, this can be very efficient in specific fish and amphibians (Poss, 2010). Even amongst animals of the same species, such as Astyanax mexicanus a teleost fish like the zebrafish, regenerative capacity can vary significantly (Stockdale et al., 2018). A better understanding of these differences and the cellular and molecular processes during tissue regeneration might ultimately help to improve the regenerative capacity of organs and tissues with poor intrinsic regenerative capacity. The mammalian adult heart has very little regenerative capacity. The myocardium lost after an injury is replaced by scar tissue, which does not contribute to cardiac contraction resulting in reduced pumping efficiency and ultimately heart failure. Although a low level of cardiomyocyte turnover has been observed, there is no evidence of a stem cell population in the mammalian heart (Kretzschmar et al., 2018; van Berlo et al., 2014). Instead, a rare population of endogenous cardiomyocytes retains the capacity to proliferate to maintain cardiac homeostasis (Bergmann et al., 2009; Bergmann et al., 2015; Kimura et al., 2015; Senyo et al., 2013). Contrary to the adult heart, the neonatal mouse and potentially human heart still have the capacity to regenerate after injury (Haubner et al., 2016; Porrello et al., 2011). This regenerative capacity of the neonatal heart involves proliferation of pre-existing cardiomyocytes and is lost soon after birth most likely due to a sharp decrease in cardiomyocyte proliferation (Porrello et al., 2011; Soonpaa et al., 1996). Recent efforts to enhance proliferation of cardiomyocytes by either inhibiting Hippo signaling or activating the Nrg1/ErbB2 signaling pathway in the adult mammalian heart have been successful and show the potential of existing cardiomyocytes to reenter the cell cycle. (Bersell et al., 2009; D'Uva et al., 2015; Heallen et al., 2011). The zebrafish heart regenerates very efficiently and will regrow cardiac muscle without scarring (Poss et al., 2002). Lineage tracing experiments revealed a model in which proliferation of preexisting cardiomyocytes replaces the myocardium that was lost during the injury (Jopling et al., 2010; Kikuchi et al., 2010). While cardiomyocyte proliferation in the uninjured adult zebrafish heart is very low, 3 days after the injury (dpi) cardiomyocytes near the injury area (so-called border zone) start to reenter the cell cycle as observed by the induction PCNA expression, phosphorylated histone three and BrdU incorporation and proliferation peaks at 7 days post injury (dpi) (Jopling et al., 2010; Kikuchi et al., 2010; Chablais et al., 2011; González-Rosa et al., 2011; Schnabel et al., 2011; Wu et al., 2016). At the time when proliferation is observed, cardiomyocytes located in the border zone start to express cardiac transcription factors known for their role in embryonic heart development such as nkx2.5 and tbx20 and activate gata4 enhancer elements (Kikuchi et al., 2010; Lepilina et al., 2006). In addition, border zone cardiomyocytes show signs of dedifferentiation such as disorganization of sarcomere structures and the reexpression of embryonic myosins (Jopling et al., 2010; Wu et al., 2016). There is increasing evidence that other (non-muscle) cells in the heart secrete growth factors that stimulate cardiomyocyte proliferation including retinoic acid, TGF-b ligands, insulin-like growth factor, Hedgehog, and Neuregulin (Chablais and Jazwinska, 2012; Choi et al., 2013; Dogra et al., 2017; Gemberling et al., 2015; Lepilina et al., 2006; Wu et al., 2016; Zhao et al., 2019; Zhao et al., 2014). In addition to these growth factors, prolonged hypoxia stimulates cardiomyocyte proliferation (Jopling et al., 2012; Marques et al., 2008). The proliferating cardiomyocytes exist within a heterogeneous cell population including non-proliferating cardiomyocytes, endothelial cells and immune cells, hampering the discovery of genetic programs specific for these proliferating cardiomyocytes using whole tissue or spatially resolved RNA-sequencing (RNA-seq) approaches (Kang et al., 2016; Lien et al., 2006; Sleep et al., 2010). To identify molecular processes that differ between proliferating and non-proliferating cardiomyocytes, we explored a single-cell RNA-seq approach using the regenerating zebrafish heart. We found that upon injury, adult border zone cardiomyocytes dedifferentiate and resemble embryonic cardiomyocytes on a transcriptomic level. In addition, while adult cardiomyocytes mainly rely on fatty acid metabolism and mitochondrial oxidative phosphorylation (OXPHOS), border zone cardiomyocytes have reduced mitochondrial OXPHOS activity while genes encoding enzymes for glycolysis are induced and glucose uptake is enhanced. Importantly, Nrg1/ErbB2 signaling is sufficient to induce metabolic reprogramming in adult cardiomyocytes of both zebrafish as well as the murine hearts. In addition, the metabolic reprogramming from mitochondrial OXPHOS to glycolysis is required for efficient cardiomyocyte proliferation. Together, these data support a model in which cardiomyocytes located in the border zone of the regenerating zebrafish heart undergo metabolic reprogramming, which is essential for cardiomyocyte proliferation and that this mechanism is conserved in a murine model with Nrg1/ErbB2 induced regeneration. Results Single-cell RNA-seq reveals transcriptionally distinct border zone cardiomyocytes The border zone comprises only a small fraction of the total number of cardiomyocytes in the injured ventricle (Wu et al., 2016). Several genes and regulatory sequencing have been identified that mark border zone cardiomyocytes, including nppa, which encodes for the cardiac stress hormone ANF (Kikuchi et al., 2010; Wu et al., 2016). To mark these borderzone cardiomyocytes we generated a transgenic zebrafish nppa reporter line (TgBAC(nppa:mCitrine)) in which mCitrine expression recapitulates endogenous cardiac expression of nppa (Figure 1—figure supplement 1a–e). While low nppa:mCitrine expression was observed in trabecular cardiomyocytes of the remote area, higher expression was detected in the trabecular and cortical cardiomyocytes close to the injured area (Figure 1a and Figure 1—figure supplement 1e). Moreover, expression of nppa correlates with previously reported border zone activity of gata4 regulatory elements (Figure 1—figure supplement 1f) (Kikuchi et al., 2010). Histochemical analysis of cryo-injured adult hearts revealed that 75% (±7%, n = 3) of the cardiomyocytes expressing high levels of nppa:mCitrine reentered the cell cycle (Figure 1a). To obtain border zone (proliferating) and remote (non-proliferating) cardiomyocytes from the same tissue for further analysis, we cryo-injured nppa:mCitrine hearts followed by cell dissociation and FACS sorting for both mCitrinehigh and mCitrinelow cells (Figure 1b). Individual, living cells were sorted, followed by single-cell mRNA-sequencing using the SORT-seq (SOrting and Robot-assisted Transcriptome SEQuencing) platform (Muraro et al., 2016) (Figure 1—source data 1). In total 768 cells where sequenced in which we detected 19257 genes. We detected an average of 10,443 reads per cell and we introduced a cutoff at minimally 3500 reads per cell before further analysis, which resulted in the analysis of 352 cells. To identify the cardiomyocytes amongst the other cell types, we first identified the different cell types based on their transcriptomes. k-medoids clustering of the single cell transcriptomes by the RaceID clustering algorithm was used (Grün et al., 2015) (Figure 1c and Figure 1—source data 2), and visualized in two dimensions using t-distributed stochastic neighbor embedding (t-SNE) (Figure 1d). A total of 12 cell clusters were identified, including a large group of cardiomyocytes (clusters 1,2,4,7 and 9), a smaller group of endothelial cells (clusters 5,6,8,10 and 12), and some fibroblasts (cluster 3) and immune cells (cluster 11) using the expression of marker genes for specific cell types (Figure 1—figure supplement 2). Based on the transcriptome clustering, the cardiomyocytes fell into four main transcriptionally-defined clusters (1, 2, 4 and 7), indicating that the injured heart contained subgroups of cardiomyocytes. To address whether the border zone cardiomyocytes were enriched in one of the four cardiomyocyte clusters we compared the mCitrine fluorescence intensity (recorded during FACS sorting) of the cardiomyocyte and found that the average intensity was highest in cluster 7 and lowest in cluster 2 (Figure 1—figure supplement 3). In addition, we analysed the single-cell transcriptome data for the expression of nppa and compared this to the expression of vmhc and mustn1b, which mark border zone cardiomyocytes, and again found that cells expressing these genes were mostly in cluster 7 (Figure 1e and Figure 1—figure supplement 4). Together, these results indicate two things: first, the border zone cardiomyocytes (grouped in cluster 7) can be identified as a separate group in the single-cell RNA-seq data. Secondly, these border zone cardiomyocytes are transcriptionally distinct from remote cardiomyocytes (grouped in cluster 2), while two intermediate cardiomyocyte clusters lie in between. Figure 1 with 4 supplements see all Download asset Open asset Single-cell mRNA sequencing identifies different cardiomyocyte-populations in the injured zebrafish heart. (a) Schematic of cryoinjury procedure on adult TgBAC(nppa:mCitrine) fish and immunohistochemistry on section of injured TgBAC(nppa:mCitrine) heart 7 dpi. Overview image on left and zoom-in of boxed region on the right. Mef2 (in magenta) labels cardiomyocytes, nppa:mCitrine (in green) marks the borderr zone, and PCNA (in cyan) marks proliferating cells. Arrows indicate triple-positive cells. Dashed line indicates injury site. Scale bar in overview 50 μm. Scale bar in zoom-ins 20 μm. (b) Experimental outline of the single-cell mRNA-sequencing of injured zebrafish hearts (blue, injury area; green, border zone) (c) Pairwise correlation between individual cells across all genes detected. Color-code indicates cell-to-cell distances measured by [1 – Pearson’s correlation coefficient]. StemID clusters are indicated by color and number on the x- and y-axis. (d) t-distributed stochastic neighbor embedding (tSNE) map representation of transcriptome similarities between individual cells. (e) tSNE maps visualizing log2-transformed read-counts of the border zone marker genes nppa, mustn1b and vmhc. Figure 1—source data 1 Single-cell mRNA sequencing data from cryoinjured TgBAC(nppa:mCitrine) zebrafish hearts at 7 dpi. Raw reads are represented per gene (rows) and single cell (columns). https://cdn.elifesciences.org/articles/50163/elife-50163-fig1-data1-v2.xlsx Download elife-50163-fig1-data1-v2.xlsx Figure 1—source data 2 List of the clusters in the adult injured heart (raw data see SD1), as identified by StemID and the associated cells per cluster. https://cdn.elifesciences.org/articles/50163/elife-50163-fig1-data2-v2.xlsx Download elife-50163-fig1-data2-v2.xlsx Figure 1—source data 3 List of differentially expressed genes between cardiomyocytes clusters 2 and 7 of the adult heart dataset. Only genes with a p-value<0.05 are listed. Per gene, the log2 fold change, adjusted p-value (padj) and associated gene name are given. GO-terms for genes upregulated between clusters 2 and 7 (p<0.01) are listed in separate excel sheets. https://cdn.elifesciences.org/articles/50163/elife-50163-fig1-data3-v2.xlsx Download elife-50163-fig1-data3-v2.xlsx Border zone cardiomyocytes resemble embryonic cardiomyocytes Cardiomyocytes in the border zone disassemble sarcomeric structures and re-express markers of embryonic cardiomyocytes suggesting their dedifferentiation. We therefore wanted to address the level of dedifferentiation of cluster seven cardiomyocytes by comparing their transcriptome with embryonic cardiomyocytes. To obtain embryonic cardiomyocytes we performed FACS sorting on embryos expressing the cardiomyocyte specific marker Tg(myl7:GFP). Single-cell mRNA-sequencing was performed and combined with the single-cell data from the injured adult hearts (Figure 2a). The RaceID algorithm identified several cell clusters with separate clusters for the embryonic and adult cardiomyocytes (Figure 2b, c and d). Importantly, the cluster seven cardiomyocytes identified in the adult data analysis had a transcriptome that was highly similar to embryonic cardiomyocytes, as shown by pairwise correlation of the differentially expressed genes between the cardiomyocyte clusters: only 257 genes (p-value<0.01), out of 23,786 total detected genes, were differentially expressed between the embryonic and the cluster 7 (border zone) adult cardiomyocytes (Figure 2d and Figure 2—source datas 1, 2, 3 and 4) suggesting a dedifferentiation of border zone cardiomyocytes to embryo--like cells. In contrast, over 1000 genes (p-value<0.01) were differentially expressed between embryonic and cluster 2 (remote zone) adult cardiomyocytes. A heatmap with unbiased hierarchical clustering on the 500 most differentially expressed genes between the three clusters confirmed that cluster 7 cardiomyocytes were more closely related to embryonic than cluster 2 cardiomyocytes (Figure 2e). Corroborating the observation that border zone cardiomyocytes resemble embryonic cardiomyocytes we found that genes encoding sarcomere proteins and cardiac-specific factors highly expressed in the embryo were re-expressed in cluster 7 (border zone) cardiomyocytes (Figure 2f, g and h). Figure 2 Download asset Open asset Single-cell transcriptome of border zone cardiomyocytes resembles that of embryonic cardiomyocytes. (a) Cartoon to illustrate the experimental procedure for single cell analysis of embryonic and adult cardiac cells. (b) tSNE map of combined adult (red) and embryonic datasets (light blue). (c) tSNE map indicating the different cell types based on marker gene expression. (d) tSNE map with the adult cardiomyocytes of the injured heart (cluster 7 in red, cluster 2 in blue and clusters 1 and 4 in gray) and embryonic (2 dpf) cardiomyocytes (in green), with number of pairwise differentially expressed genes (p-value<0.01) indicated between cardiomyocyte clusters. (e) Heatmap with hierarchical clustering based on the 500 most differentially expressed genes between clusters. Red color represents high expression, blue color represents low expression. Rows represent individual genes. (f–h) tSNE maps visualizing log2-transformed read-counts of vmhc (f), nppa (g) and tnnt2a (h). Figure 2—source data 1 Single-cell mRNA sequencing data from Tg(cmlc2:GFP) zebrafish hearts at 2 days post fertilization. Raw reads are represented per gene (rows) and single cell (columns). https://cdn.elifesciences.org/articles/50163/elife-50163-fig2-data1-v2.csv Download elife-50163-fig2-data1-v2.csv Figure 2—source data 2 List of the clusters from the embryonic heart data (SD5) identified by StemID and the associated cells for each cluster. https://cdn.elifesciences.org/articles/50163/elife-50163-fig2-data2-v2.xls Download elife-50163-fig2-data2-v2.xls Figure 2—source data 3 Combined single-cell mRNA sequencing data from embryonic and adult hearts. Raw reads represented per gene (rows) and single cell (columns). https://cdn.elifesciences.org/articles/50163/elife-50163-fig2-data3-v2.csv Download elife-50163-fig2-data3-v2.csv Figure 2—source data 4 List of pairwise differentially expressed genes between all cardiomyocyte clusters from the combined embryonic and adult datasets. Only genes with a p-value<0.01 are listed. Per gene, the log2 fold change, adjusted p-value (padj) and associated gene name are given. GO-terms for genes upregulated between clusters 5 and 6 (p<0.01) are listed in separate excel sheets. https://cdn.elifesciences.org/articles/50163/elife-50163-fig2-data4-v2.xls Download elife-50163-fig2-data4-v2.xls To identify cellular events that occur during this dedifferentiation we used part of the RaceID algorithm (StemID) that uses the single cell transcriptome data and cell clustering to derive a branched lineage tree (Grün et al., 2016). The algorithm is based on the premise that stem cells and less differentiated cells tend to exhibit more uniform transcriptomes than differentiated cells, which express smaller numbers of genes at higher rates (Banerji et al., 2013). Using this approach, we found large differences in transcriptome entropy, resulting in low (cluster 2), intermediate (clusters 1 and 4) and high (cluster 7) StemID scores (Figure 3a). This gradual increase suggests a dedifferentiation axis from cells in cluster 2 (remote myocardium) to cells in cluster 7 (border zone myocardium) and is in good agreement with our finding that the transcriptome of cluster 7 cardiomyocytes resembles an embryonic cardiomyocyte transcriptome (Figure 3b). Together, these results indicate that clusters 4 and 7 are enriched for dedifferentiated and proliferative border zone cardiomyocytes while clusters 1 and 2 are enriched for differentiated remote cardiomyocytes. Figure 3 with 1 supplement see all Download asset Open asset Pseudo time analysis reveals dedifferentiation and metabolic changes in border zone cardiomyocytes. (a) Bar plot of StemID scores for the cardiomyocyte clusters (clusters #2, 1, 4 and 7) calculated by the formula: number of significant links for each cluster multiplied by the median transcriptome entropy across all cells in a cluster. (b) Cardiomyocyte clusters from adult injured heart. Arrow indicates the dedifferentiation path derived from the StemID scores. (c) Pseudo time analysis. Left; one-dimensional SOM of z-score transformed expression profiles along the differentiation trajectory incurred by StemID analysis. Y-axis represents the fourteen modules with differentially expressed genes. X-axis represents the pseudo time in which the cells were ordered. Middle; expression profiles of representative genes of the major modules. Y-axis shows transcript counts. X-axis represents the pseudo time. Right; Major gene ontology terms derived from all genes expressed in the module with p-values. Figure 3—source data 1 List of genes that are differentially upregulated in the respective modules identified in the pseudo timeline analysis (related to Figure 3c). https://cdn.elifesciences.org/articles/50163/elife-50163-fig3-data1-v2.xlsx Download elife-50163-fig3-data1-v2.xlsx Border zone cardiomyocytes induce glycolysis, which is required for proliferation While cardiomyocytes undergo a well-defined sequence of morphological and transcriptional changes during differentiation, very little is known about the reverse process. Ordering whole-transcriptome profiles of single cells with an unsupervised algorithm can resolve the temporal resolution during differentiation by identifying intermediate stages of differentiation without a priori knowledge of marker genes (Trapnell et al., 2014). In this manner, the single-cell mRNA-seq experiment will constitute an in-silico time series, with each cell representing a distinct state of differentiation along a continuum. To analyze the transcriptional changes occurring during this apparent dedifferentiation, the most likely dedifferentiation path, based on the StemID scores, was chosen starting at cluster two and progressing through clusters 1, 4 and 7. Next, gene expression profiles along this pseudo-temporal order were computed for all detected genes using the single-cell transcriptomes. These gene expression profiles were grouped into modules of co-expressed genes using self-organizing maps (SOMs), resulting in 14 modules (Figure 3c, Figure 3—source data 1). Corroborating our hypothesis of varying differentiation states, we observed that gene expression within these modules changed smoothly over pseudo time. We next analyzed the temporally-ordered expression profiles and identified four groups of genes that shared the same dynamics of expression during this differentiation trajectory. The first group (modules 1, 2) contained genes that were most highly expressed only in cells at the very beginning of the pseudo time line and their expression rapidly declined in cells that were positioned later. This group contained many genes transcribed from mitochondrial DNA and with a role in energy metabolism, which indicates that the cells at the start of the pseudo time line are mature cardiomyocytes. The second group (module 6) contained genes that are induced early and expression stayed constant in cells further along the pseudo time line. Many genes involved in translation and cell cycle regulation follow this expression pattern. The third group showed an exponential increase in expression with the highest expression at the end of the pseudo time line (module 10). This group contained genes with a function in cardiac muscle fiber development and heart contraction. The fourth group (modules 11 and 14) contained genes with a rapid increase in expression that peaks before the end of the pseudo time, suggestive for an early role during the dedifferentiation process. Interestingly this group contained many genes with a known role in glycolysis. Together, these data suggest that border zone cardiomyocytes undergo profound metabolic changes. This was confirmed by GO-term analysis between cluster 7 and cluster 2 cells (Figure 4—figure supplement 1a and b, Figure 1—source data 3). To validate the functional consequences of the observed changes in mitochondrial gene expression, we measured succinate dehydrogenase (SDH) enzyme activity, located in the inner mitochondrial membrane that functions in both the citric acid cycle and electron transport chain. We observed a 40% reduction in SDH activity specifically in the border zone cardiomyocytes as compared to the remote cardiomyocytes (Figure 4a). In agreement with the reduced mitochondrial OXPHOS activity, transmission electron microscopy (TEM) imaging revealed more immature mitochondria in border zone cardiomyocytes evidenced by their altered morphology and reduced cristae density (Figure 4b), which is consistent with previous reports linking mitochondrial function with morphology (Giraud et al., 2002; Paumard et al., 2002). Since the pseudotime analysis suggested an upregulation of glycolytic gene expression in the border zone cluster (#7), we performed gene set enrichment analysis (GSEA) for glycolysis genes. The GSEA revealed a strong and significant enrichment in the expression of glycolytic genes in cluster 7 cells compared to cluster 2 cells (Figure 4—figure supplement 1c). By in situ hybridization we confirmed the induced expression in border zone cardiomyocytes of the rate-limiting enzymes hexokinase (hk1), pyruvate kinase M1/M2a (pkma) and pyruvate dehydrogenase kinase (pdk2a), which diverts pyruvate away from the TCA cycle (Figure 4c and Figure 1—figure supplement 4b). Corroborating the suggested enhanced glycolysis, we observed induced expression of glucose importer genes (glut1a/slc2a1a and glut1b/slc2a1b) in cluster 7 cells (Figure 4—figure supplement 1d) and enhanced in vivo glucose uptake of border zone cardiomyocytes (Figure 4d). Furthermore, genes encoding lactate transporters and their proteins were upregulated in cluster seven and border zone cardiomyocytes (Figure 4e and Figure 4—figure supplement 1d). Together, these data indicate that during regeneration border zone cardiomyocytes switch energy metabolism from mitochondrial OXPHOS to glycolysis and lactate fermentation. Figure 4 with 1 supplement see all Download asset Open asset Border zone cardiomyocytes undergo a metabolic switch from mitochondrial OXPHOS to glycolysis. (a) Succinate dehydrogenase (SDH) enzyme staining on a seven dpi heart section with injury area separated by dashed line. Quantification of SDH activity in remote zone (RZ), border zone (BZ) and injury area (IA). Scale bar indicates 100 μm. Error bars indicate mean and standard deviation. (b) Transmission electron microscopy (TEM) images of mitochondria in cardiomyocytes from the remote zone and the border zone of a 7 dpi injured heart. Note the disorganized and irregular shaped mitochondria in the border zone cardiomyocyte. Scale bar 500 nm (200 nm in inserts). Graphs show quantification of mitochondrial perimeter-to-area as a measurement for roundness and quantification of mitochondrial cristae density. * p-value<0.05. (c) In situ hybridizations for glycolytic genes hk1, pkma and pdk2a on sections of injured zebrafish hearts at 7 dpi. Dashed line indicates injury site. Scale bars indicate 100 μm. (d) Time-lapse multi-photon confocal images of whole heart. The heart was isolated at 7 dpi and incubated with 2-NBDG, a fluorescent glucose analogue, at t = 0. Dotted line indicates injury area. Arrows point to regions of the border zone. Scale bar represents 100 μm. (e) Confocal image of injured zebrafish hearts at 7 dpi stained for the lactate transporter MCT4 (green) and Tropomyosin (red). Dashed line indicates injury site. The pseudo time line analysis suggested that glycolysis genes induction precedes the induction of embryonic cardiac gene expression. Indeed, ISH analysis showed that glycolysis gene expression is already induced at 3 dpi and thereby precedes expression of embryonic cardiac gene expression and cardiomyocyte proliferation, which peaks at 7 dpi (Figure 3—figure supplement 1). To address the functional importance of glycolysis we inhibited glycolysis in injured fish with the glucose analogue 2-Deoxyglucose (2-DG), a general inhibitor of glycolysis, and analyzed its effect on cardiomyocyte proliferation (Figure 5a). We observed that repeated injections of 2-DG in the adult zebrafish with a cryoinjured heart significantly impaired cardiomyocyte proliferation in the border zone (Figure 5b,c), suggesting that glycolysis is necessary for cell cycle reentry. Figure 5 Download asset Open asset 2-Deoxy glucose impairs cardiomyocyte proliferation. (a) Experimental design for the 2-DG injections to inhibit glycolysis in injured zebrafish hearts. (b) Confocal image of injured zebrafish hearts at seven dpi either injected with PBS or 2-DG stained for Mef2c (green) and PCNA (magenta). Zoom-in images of the borderzone are shown below overview pic
更多查看译文
关键词
cardiomyocyte proliferation,erbb2,metabolic,single-cell
AI 理解论文
溯源树
样例
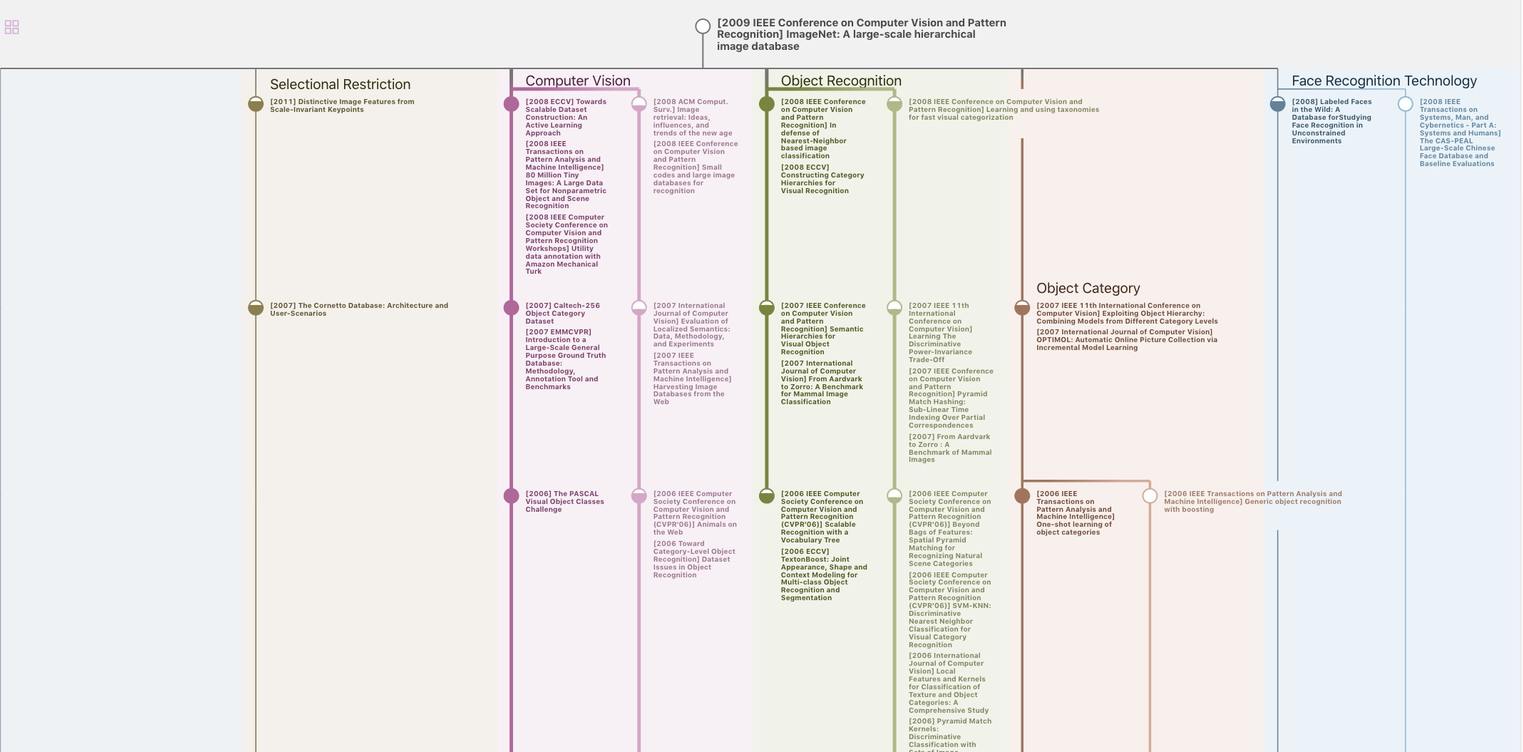
生成溯源树,研究论文发展脉络
Chat Paper
正在生成论文摘要