A Distributed Electrical Network to Model the Local Shunting in Multicrystalline Silicon Solar Cells
world conference on photovoltaic energy conversion(2012)
摘要
In this paper we propose a three-dimensional (3-D) distributed electrical circuit for the modeling of solar cells. The developed tool is based on a network of repetitive elementary cells, each modeled by a two-diode electrical circuit and allows to account for the transport through the emitter, the fingers and the busbars. Moreover, the tool is able to account for the non-uniformity in the solar cell. In order to validate our tool, we calibrate the electrical parameters according to experimental measurements on multicrystallinesilicon (mc-Si) solar cells. By using the thermographic analysis, we detect hot spot regions inside the mc-Si devices and we model them by means of local shunting in our tool. The presence of local shunting, due to localized crystal defects, results in a degradation of the open-circuit voltage, of the fill factor and then of the overall power conversion efficiency. Purpose of the work and approach Multicrystalline-silicon (mc-Si) is a commercially important material for manufacturing of solar cells. However, the efficiency of mc-Si solar cells is limited by the presence of material defects. Intra-grain dislocations and impurity precipitates represent the main sources of local shunting [1]. The purpose of this work is to demonstrate the capabilities of a 3-D distributed electrical circuit that allows to accurately describe the electrical performance of silicon solar cell in case of non-uniformity along the substrate. First, we validate our tool with experimental measurements on mc-Si solar cells and with two-diode lumped model (which assumes a uniform distribution of parameters in the substrate). Then, we analyze the behavior of mc-Si solar cells under different illumination levels, considering the presence of local defects with different spatial distribution on the solar cell area. The analysis is done by using both experimental measurements and circuit simulations. For the experimental characterization, three types of measurements are considered: dark I-V characterization, thermographic characterization and I-V curves at different light levels using a LED-based solar simulator. Scientific innovation and relevance The understanding of the influence of local shunting in mc-Si solar cell performance is a relevant issue in the photovoltaic community. It is very important to model the spatial distribution of defects in mc-Si solar cells in order to understand the impact on the solar cell efficiency. Our distributed electrical model provides detailed information on the spatial distribution of voltage and current over the whole cell area. A good agreement can be appreciated from the comparison between experimental measurements and simulation results, obtained with the proposed model. This reveals the potentiality of the model to predict the behavior of solar cell with detailed information about crystal defects, materials inhomogeneities or dislocations. This can be very helpful to improve the performance of the next generation of wafer-based silicon solar cells. Results and Conclusions In this work we have modeled the effect of local shunting on mc-Si solar cell by means of a three-dimensional distributed SPICE network. The electrical parameters of the distributed electrical network are calibrated on the basis of experimental measurements of two representative samples of mc-Si solar cells featuring low and high shunt resistance. Infrared thermographic characterization clearly reveals how the shunt resistance is strongly correlated to the presence of localized defects, that contribute to the generation of hot spots. Both experimental and simulation results show significantly different performance for the two considered cells, particularly in terms of fill factor and hence of conversion efficiency. Moreover, by performing the analysis under different illumination conditions, it is possible to see that the effect of a low shunt resistance is more relevant at low irradiation levels. Finally, the developed distributed electrical model is exploited to understand how the different spatial distribution of local defects can impact on the solar cell performance. The hot spot area has been modeled with a much lower shunt resistance, with respect to the remaining of the cell area. We observed that, by modeling this low specific shunt resistance as the measured overall shunt resistance (0.26Ω) times the hot spot area (1.6cm), simulated results are strongly different from experimental measurements. In particular, higher value of fill factor and efficiency are found. Therefore, a lower value of specific shunt resistance must be considered. By using specific shunt resistance for the hot spot area much below 1mΩcm, a good agreement between experimental measurements and simulation is achieved. This analysis clearly reveals that the overall shunt resistance is an equivalent value and does not give information on the local shunting phenomena. On the other hand, the distributed electrical network, correlated with the thermographic map, allows to understand in more detail and to properly model the local shunting in the solar cell. Figure 1. Elementary units implemented in the distributed electrical network. They are based on the twodiodes model. The elementary unit (a) is suitable to model the area under the fingers and the busbars. The elementary unit (b) allows to model the area under the non-metalized regions. (a) (b) 1 DESCRIPTION OF DISTRIBUTED ELECTRICAL NETWORK In the proposed model we represent a solar cell by means of a resistive network which connects two-diode equivalent circuits. In Fig. 1a and Fig. 1b we report two types of elementary unit. They allow to model the solar cell properties under the metalized regions and under the non-metalized regions, respectively. Both elementary units are composed by distributed resistances (RE) to account for the transport through the emitter region, and by two diodes to describe the recombination currents of the pn-junction. The shunt resistance RSH is placed in parallel to the two diodes. In particularly, the currents ID1 and ID2 are given by: ID1 (x,y)=A(x,y)J01⋅[exp(V(x,y) /η1VT -1] (1) ID2(x,y) = A(x,y)J02⋅[exp(V(x,y)/η2VT -1] (2) where η1, η2 are the ideality factors, VT is the thermal voltage and A(x,y) is the area of the elementary unit. An ideal current source is implemented in order to account for the photogenerated current (IPH), which is supposed to be proportional to the solar irradiation G [W/m]. The contact resistances of fingers and the busbars, are modelled with the resistor RC, while RM represents the resistance due to the conduction through the metal structures (i.e. fingers and busbars). Each of the above mentioned parameters depends on the position (x,y) inside the solar cell. Therefore, the tool allows to account for non-uniformity in the cell parameters. It is worth noting that the elementary units are slightly modified on along the perimeter regions in order to account for the boundaries of the cell. Finally, the whole solar cell can be modeled by opportunely interconnecting the elementary units. The proposed distributed network can be used to model the behavior of solar cell in both dark and illuminated conditions. In particularly, the dark analysis can be easily performed by forcing IPH = 0. The distributed electrical network must be solved by using a circuit simulator like SPICE. In this work we adopt LTSpice from Linear Technology Corporation. 2 EXPERIMENTAL DETAILS In order to highlight how material defects influence the behavior of the cells, we take two representative samples of mc-Si solar cells with high and low shunt resistance, respectively. The main solar cell parameters are obtained using three typologies of measurements: Dark I−V characterization, Thermographic characterization and I−V curves at different light levels using a LED-based solar simulator. In the following we describe our measurement setup. Using a source meter we made a dark I-V characterization of the two cells with different levels of shunting. The measurements were carried out with a four wire technique in order to minimize the effect of the parasitic impedance of the cables. The different shunt resistances of the cells are clearly recognizable in the low voltage region of the curves (Fig. 4). In order to evaluate the presence of hot spots in reverse bias, we carried out infrared thermographic measurements. Within this work, we have realized a setup for “synchronous-pulsed” thermal characterization, that allows for a better spatial resolution, without the need of a more expensive Lock-in thermographic system [6]. Fig. 2 shows a schematic block diagram of the measurement setup: a current pulse is applied on the cell for 500 ms in reverse bias conditions and a spatially resolved thermographic image is acquired immediately after the voltage on the cell has stabilized. Then a zero current is applied for about ten seconds, to cool down the cell, and then another measurement can be taken. The final image is obtained as the average of over ten images in order to reduce noise. This kind of measurement setup is used to reduce selfheating of the cell during the measurements, thus increasing spatial resolution. Figure 2. Schematic diagram of spatially resolved thermography measurement set up. Thermo-‐ Camera Source Meter PC LabView
Control Thermography
acquisitions 10
s 0.5
s LabView
Control
更多查看译文
关键词
simulation
AI 理解论文
溯源树
样例
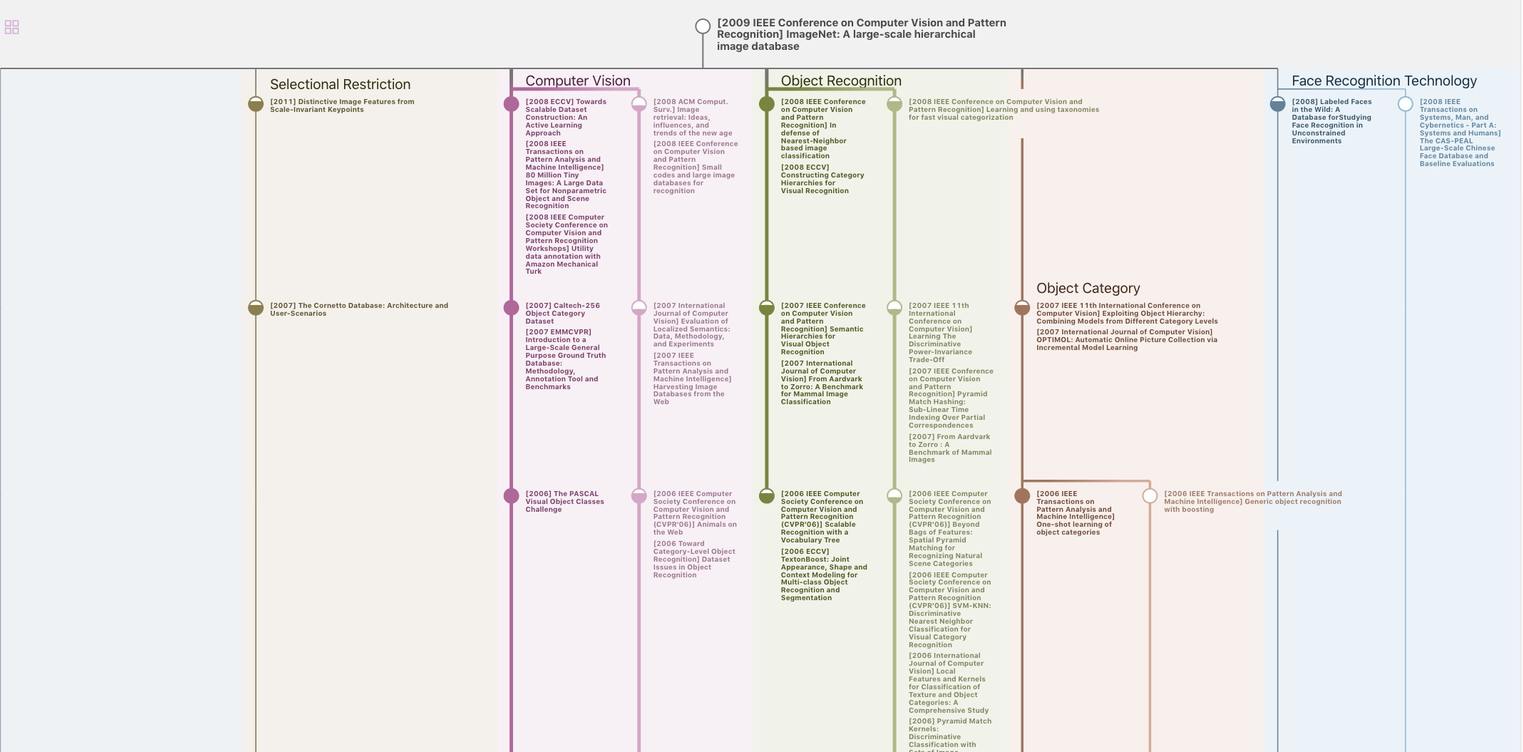
生成溯源树,研究论文发展脉络
Chat Paper
正在生成论文摘要