Enzyme-catalysed [lsqb]4+2[rsqb] cycloaddition is a key step in the biosynthesis of spinosyn A
Nature(2011)
摘要
Spinosyn A (1), an active ingredient of several highly effective and environmentally benign commercial insecticides, has a complex aglycone structure comprising a perhydro-as-indacene moiety fused to a 12-membered macrolactone13, 14. How this tetracyclic ring system is biosynthesized has been a subject of much speculation16, 17, 18. Attention has largely focused on the construction of the cyclohexene ring due to the potential involvement of an enzyme that catalyses the [4+2] cycloaddition, which if concerted would represent a so-called ‘Diels–Alderase’. Four genes in the spinosyn A biosynthetic gene cluster of S. spinosa—spnF, spnJ, spnL and spnM—were proposed to convert product (2) of the polyketide synthase (PKS) to the tetracyclic aglycone (4) (see Fig. 1)16. The gene product of spnJ, which is a flavin-dependent dehydrogenase, was recently demonstrated to catalyse oxidation of the 15-OH of 2 to form the keto-intermediate 3 (ref. 19). However, the functions of the enzymes encoded by the remaining three genes, spnF, spnL and spnM, which show significant sequence similarity to lipases (SpnM) and S-adenosyl-l-methionine (SAM)-dependent methyltransferases (SpnF and SpnL)16, remain elusive. To investigate the functions of SpnF, SpnL and SpnM, the corresponding genes were heterologously overexpressed in Escherichia coli BL21(DE3)* and their products purified as N-terminal His6-tagged proteins (>95% purity). Each of the purified enzymes was tested for activity with 3. As shown in Fig. 2A, neither SpnF nor SpnL processes 3. In contrast, complete conversion of 3 to a new product was observed after a 2-h incubation with SpnM. NMR and mass spectrometry analysis of this new product (8) revealed a 15,6,5-tricyclic skeleton. Further investigation of the reaction time-course led to the discovery of a transient intermediate (Fig. 2D, orange peak), which was identified by spectral analysis as the monocyclic macrolactone 5. These findings indicated that the SpnM-catalysed conversion of 3 to 8 might be a two-step process involving 1,4-dehydration of 3 followed by a transannular [4+2] cycloaddition between the Δ11,12-alkene and the conjugated Δ4,5,Δ6,7-diene of intermediate 5 to form the cyclohexene moiety in 8 (Fig. 4). To investigate whether SpnM is indeed a cyclase of dual function catalysing both the dehydration and cyclization steps, the dependence of the rate of each step on the concentration of SpnM was determined. In this experiment the formation and consumption of 5, which respectively reflect the dehydration and cyclization steps, was monitored by high-performance liquid chromatography (HPLC) as a function of time. As shown in Fig. 3a, rate enhancement of the dehydration step was observed with the increase in SpnM concentration, whereas the rate of cyclization was unaffected. The full time courses of the formation and decay of 5 were analysed using numerically integrated coupled rate equations based on different kinetic models (see Supplementary Information Section 5)20. The observed data was fit best to the integrated rate equations (1a) and (1b), which model Michaelis–Menten kinetics for the dehydration step (VSpnM and KSpnM) and first order kinetics (knon) for the cyclization step. Correlation of the fitted parameters versus the concentration of SpnM (Fig. 3b) reveals a significant dependence of VSpnM, but not KSpnM or knon, on SpnM concentration. This analysis indicates that SpnM functions only as a dehydratase, and its product (5) can cyclize nonenzymatically to 8 with a first order rate constant of approximately 0.03 min−1. Having established that SpnM functions as a dehydratase, whereas the [4+2] cycloaddition can proceed nonenzymatically, we next considered the possible involvement of the two remaining gene products, SpnF and SpnL, in the final C-C bond formation between C-3 and C-14. Surprisingly, incubation of SpnF or SpnL with 8, which was generated in situ from 3 in the presence of SpnM, did not produce the aglycone 4 (Fig. 2B). However, when SpnF was added to the assay mixture, a change in the product distribution was noted including rapid disappearance of 5 (Fig. 2B, trace c). As shown in Fig. 2E, the consumption of 5 with concomitant formation of a product having a retention time consistent with 8 was completed in 20 min in the presence of 10 μM SpnF, instead of requiring more than 2 h in its absence (Fig. 2D). The structure of this product was established to be 8 by NMR analysis. Additional controls, including examining the effects of denatured SpnF as well as mutated SpnF on the rate of cyclization, were also performed. In all cases only native (His6-tagged) SpnF led to appreciably increased rates of cyclization (see Supplementary Information Section 4.4). These results clearly indicate that SpnF is responsible for the observed rate enhancement of the cyclization of 5 to 8. To quantify the effect of SpnF on the rate of the cyclization step, the production and consumption of 5 was again monitored by HPLC, this time as a function of SpnF concentration while keeping that of SpnM fixed. As shown in Fig. 3c, the rate of conversion of 5 to 8 is clearly enhanced as the concentration of SpnF increases. The data was fit best using the coupled rate equations (2a) and (2b), where the cyclization event is modelled as the sum of a first order and a Michaelis–Menten process. In these fits all parameters including the initial concentration of 3 were allowed to float except for knon, which was fixed at the fitted value obtained in the absence of SpnF. Correlation of the fitted parameters with SpnF concentration indicates a significant dependence of VSpnF on SpnF concentration (Fig. 3d), which is not true for the VSpnM parameter. These results firmly establish that SpnF is a cyclase catalysing the conversion of 5 to 8 with an apparent kcat of 14 ± 1.6 min−1 (± s.e.) for an estimated rate enhancement (kcat,spnF versus knon) of approximately 500-fold. This result left spnL as the only gene without an assigned function, and it was proposed that SpnL has a role in the transannular cyclization reaction between C-3 and C-14. However, the observed inability of SpnL to convert 8 to the anticipated product (4) (Fig. 2B, trace b) prompted us to reconsider the sequence of events of the proposed biosynthetic pathway in Fig. 1. Previously, glycosylations had been thought to occur on the tetracyclic aglycone (4), because transfer of the rhamnose moiety from thymidine diphosphate-β-l-rhamnose (TDP-β-l-rhamnose, 9) to 9-OH of 4 by the rhamnosyltransferase, SpnG, had been demonstrated21, 22. However, this observation did not necessarily rule out an alternative pathway in which rhamnosylation of 8 precedes C-3/C-14 cross-bridging, because glycosyltransferases involved in the biosynthesis of secondary metabolites are known to be promiscuous with regard to their substrate specificity23, 24. To test this possibility, the ability of SpnG to accept 8 as a substrate was investigated. As shown in Fig. 2C (trace b), incubation of 8 and 9 with SpnG resulted in the disappearance of 8 with the concomitant appearance of a new peak (retention time of 17 min). This new product was identified as 10 by NMR and mass spectrometry analysis (Fig. 4). Upon addition of SpnL to this reaction mixture, the peak at 17 min disappeared and a new peak appeared at 27 min (Fig. 2C, trace c). Both transformations were highly efficient. Identification of the new product as 13 was confirmed by high-resolution mass spectrometry analysis and HPLC co-elution with an authentic sample of 13. These results strongly suggest that 10 rather than 8 is the biological substrate for SpnL, which catalyses the final cyclization step to generate the perhydro-as-indacene core. The mechanisms by which SpnF and SpnL catalyse their respective cyclization reactions are a point of interest. The SpnF-catalysed endo-mode syn-addition of an alkenyl to a dienyl functionality seems consistent with a Diels–Alder reaction; however, confirmation of this hypothesis will require demonstrating that the reaction progresses through a single pericyclic transition state such as 6. Therefore, a stepwise [4+2] cycloaddition mechanism, for example, one involving a dipolar intermediate such as 7, cannot at present be ruled out. In contrast, the C-C bond formation catalysed by SpnL may involve a Rauhut–Currier mechanism15 consistent with the observation that 10 is susceptible towards nucleophilic addition by a thiol (see Supplementary Information Section 3.10) forming a covalent adduct that may be structurally analogous to 11 or 12 (see Fig. 4), although the specific site of attack remains unknown. Whereas these mechanistic proposals are at present speculative, it is worth noting that Roush and co-workers were able to accomplish their non-enzymatic total synthesis of spinosyn A by exploiting both the transannular Diels–Alder and Rauhut–Currier reactions in an analogous fashion25. This precedent in chemical synthesis certainly substantiates the feasibility of the mechanisms proposed for the SpnF- and SpnL-catalysed reactions. In summary, the biosynthetic pathway for spinosyn A is now fully established (Fig. 4), with the specific functions of SpnM as a dehydratase and SpnF as well as SpnL as the two cyclases in the cross-bridging steps biochemically determined. SpnF represents the first enzyme for which specific acceleration of a [4+2] cycloaddition reaction has been experimentally verified as its only known function. It stands in contrast to macrophomate synthase, for which evidence has been provided suggesting a tandem Michael–aldol reaction mechanism26, 27, as well as the multifunctional solanapyrone synthase, LovB and riboflavin synthase, which participate in hydroxyl oxidation5, 6, polyketide synthesis7, 8, and hydride transfer12, respectively, in addition to the [4+2] cycloaddition reactions, the concertedness of which have yet to be verified. For this reason, the SpnF reaction provides a unique system for detailed mechanistic investigation of enzyme-catalysed [4+2] cycloadditions and the existence of a bona fide Diels–Alderase. All proteins used in this work were overexpressed in E. coli BL21(DE3)* (Invitrogen) and purified by Ni-NTA (Qiagen) affinity chromatography. Specifically, SpnF was co-overexpressed with the chaperone protein pair, GroEL/ES, to improve its solubility. Because overexpression of the protein encoded by the originally assigned spnM gene16 failed to afford an active soluble protein, the gene sequence was re-examined and revised to include 204 additional base pairs (Supplementary Fig. 2). Overexpression of the revised spnM gene produced an active enzyme with significantly improved protein yield. All enzyme reaction products (5, 8, 10 and 13) were extracted with ethyl acetate or chloroform and purified using silica gel column chromatography or HPLC. Their structures were characterized by 1D- and 2D-NMR spectroscopy and/or high-resolution mass spectrometry. In particular, the stereochemistry of 8 was assigned based on its 1H-1H nuclear Overhauser enhancement spectroscopy (NOESY) spectrum. All substrate specificity and time-course assays were run in 50 mM Tris-HCl buffer (pH 8) at 30 °C. Reaction aliquots were quenched with an excess volume of ethanol after a given incubation time and centrifuged to remove protein. The supernatant was then analysed by reverse phase HPLC with detection by ultraviolet absorbance at 254 nm (Fig. 2) or 280 nm (Fig. 3). Time course assays also included p-methoxyacetophenone as an internal standard to normalize the peak areas corresponding to 5. Numerical integration of equations (1) and (2) used the fourth order Runge–Kutta algorithm28 following non-dimensionalization of substrate concentration. The resulting simulated progress curves were fit using steepest descent29 directly to full time-courses of normalized substrate concentration obtained via the HPLC discontinuous assay to provide the kinetic parameters and a concentration normalization factor. Further detail regarding experimental procedures as well as data fitting and analysis is described in the Supplementary Information. Download references We thank C. Whitman for review of the manuscript, L. Hong for carrying out the early cloning work, B. Shoulders and S. Sorey for assistance with the interpretation of the NMR spectra, and S. Mansoorabadi and E. Isiorho for discussions on the reaction mechanisms and structural modelling of SpnF. This work is supported in part by grants from the National Institutes of Health (GM035906, F32AI082906), the Texas Higher Education Coordination Board (ARP-003658-0093-2007), and the Welch Foundation (F-1511).
更多查看译文
关键词
Chemical biology, Biochemistry, Chemistry
AI 理解论文
溯源树
样例
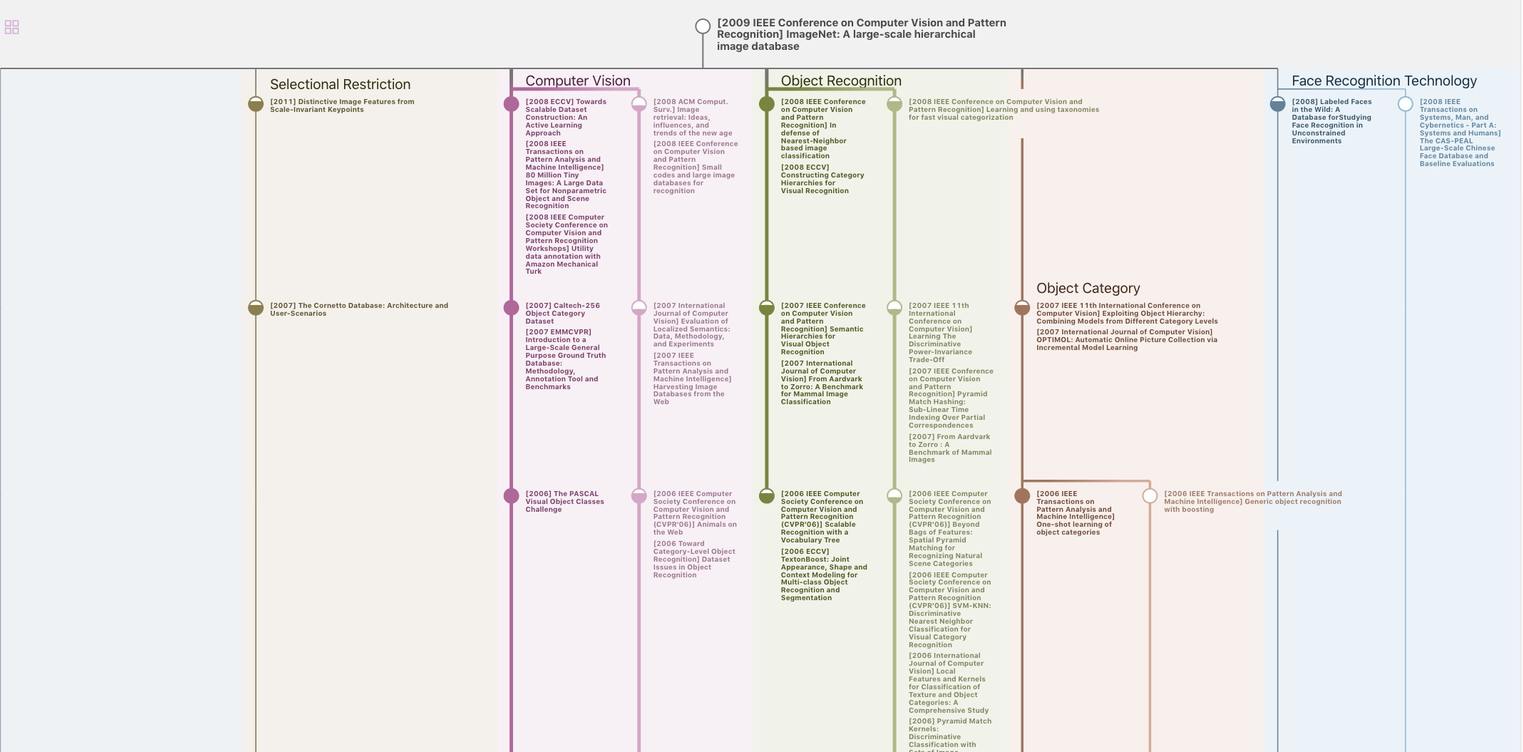
生成溯源树,研究论文发展脉络
Chat Paper
正在生成论文摘要