Two-wave mixing of ion-implanted photorefractive waveguides in near-stoichiometric Fe:LiNbO3 crystals
Optical Materials(2011)
摘要
Research highlights ► H implantation forms optical waveguides in Fe doped stoichiometric LiNbO 3 crystal. ► Two-wave mixing gain coefficient is 15 cm −1 at 632.8 nm. ► Significant shorter response time than the bulk at very low power is obtained. ► Very good photorefractive features are found in the waveguide. Abstract We report on the two-wave mixing of light in photorefractive waveguides in H ion-implanted Fe-doped near-stoichiometric lithium niobate crystals. For pump light of 632.8 nm wavelength a gain coefficient as high as 15 cm −1 is found. A response time of the order of a few seconds is achieved for micro-watt input powers. Keywords Ion implantation Stoichiometric LiNbO 3 Optical waveguides Two-wave mixing 1 Introduction Optical waveguides allow for strong confinement of light in very small cross sections, resulting in high optical intensities over large propagation distances. Consequently, the nonlinear response of bulk materials can be significantly enhanced using waveguide or fiber-like structures. Some recent examples are super-continuum generation in photonic crystal fibers [1] or efficient nonlinear frequency conversion in photonic nano-wires [2] . On the other hand, the high light intensities that are inherent to waveguide structures may also enable the speed-up of rather slow nonlinear processes like the photorefractive effect. In a photorefractive material, refractive index changes can be induced at relatively low optical power levels, which is of particular interest for applications in holographic storage and optical communications [3] . When using waveguide geometries, a better performance may be expected when compared to the bulk, e.g., higher gain factors in wave mixing and shorter response times caused by the high photoconductivity, which is proportional to light intensity [4] . In addition, the waveguides may be easily connected with micron-scale elements like optical fibers, photodiodes or semiconductor lasers. Lithium niobate (LiNbO 3 ) is a well-known single-crystalline photonic material with many applications in optoelectronics owing to its excellent combination of electro-optical, nonlinear optical, and photorefractive properties [5] . When doped with metal ions like Cu or Fe, rather large, reversible light-induced index changes of the order of 10 −4 –10 −3 can be obtained [6] . The mostly used commercially available LiNbO 3 crystals are wafers of the congruent composition (CLN), where the ratio of [Nb/(Nb + Li)] is around 48.5%. In CLN, Nb atoms on Li sites form an intrinsic, photo-active defect with very high concentration [6] , resulting in the degradation of some properties [7] . Recently near-stoichiometric LiNbO 3 crystals (SLN), which have a ratio [Nb/(Nb + Li)] > 49.5%, have received much attention owing to a significant improvement of the crystal structure as well as the related linear and nonlinear optical properties [7] . When doping rare-earth ions into SLN an improved luminescent performance has been reported [8] . Furthermore, in Fe-doped SLN an increased photorefractive sensitivity is observed when compared to Fe:CLN [9] . Standard methods for waveguide fabrication in congruent LiNbO 3 crystals are in-diffusion of Ti at high temperature [10] , or proton exchange where Li atoms are replaced by protons [11] . However, in both cases the concentration of Li is reduced in the near-surface region (in the first case at high temperatures Li diffuses out of the surface). Thus, both methods are less suitable in the case of stoichiometric substrates, where the Li concentration should be maintained close the exact stoichiometric composition. As an alternative method, ion implantation is a widely applied technique for material modifications, and has been successfully used to form low-loss optical waveguides in crystalline and non-crystalline substrate materials [12,13] . Recently, SLN waveguides have been fabricated by implantation using different ions, and guiding properties and luminescent properties have been investigated [14–16] . However, the photorefractive properties of SLN waveguides have not yet been explored. In this work, we report on, for the first time to our knowledge, waveguide formation in a Fe-doped SLN (Fe:SLN) crystal, and the demonstration of its photorefractive properties by using the two-wave mixing (TWM) method. 2 Experimental The sample used in our experiment was a x -cut stoichiometric LN crystal doped with 0.03 wt.% Fe in the melt and cut to dimensions of 1.5( x ) × 8( y ) × 10( z ) mm 3 . The crystal has a Li 2 O content of 49.97 mol% (corresponding to a Li/Nb ratio of 0.9988) that is obtained by the vapor-transport equilibration (VTE) method [17] in a Li-rich atmosphere using an initially congruent sample. One optically polished facet (with size of 8 × 10 mm 2 ) of the wafer is implanted by H + ions at energy of 480 keV and a fluence of 4 × 10 16 ions/cm 2 . After implantation, the sample is annealed at 260 °C for 30 min in air for two reasons. Firstly, after implantation the concentration of generated colour centers has to be reduced in order to increase the optical transmission. Secondly, the implantation of positively charged ions may lead also to an increase of the Fe 2+/3+ ratio, as observed in the case of cerium-doped SBN crystals [18] , and thus transmission may be decreased due to photo-excitation of electrons from Fe 2+ impurities, too. When annealing in air at moderate temperatures, oxygen diffusion into the surface layer will at least partially compensate for this possible chemical reduction effect. Furthermore, annealing also increases the general thermal stability of the implanted sample. Finally, the two facets (with size of 1.5 × 8 mm 2 ) are polished to allow for coupling light into and out of the waveguides. The m -line technique [19] has been used to measure the guided modes of the planar waveguides through a prism coupler (Metricon 2010, USA) at a wavelength of 632.8 nm. The propagation loss of the waveguide is measured to be ∼1 dB/cm at 632.8 nm. We use an end-facet coupling system to measure the TWM parameters of the waveguides (see Fig. 1 for a schematic of the experimental setup). The extraordinarily polarized light of a Helium–Neon laser at a wavelength of 632.8 nm is divided into two beams through a beam splitter, to serve as the signal and pump beams, respectively. A neutral density filter is used to control the power of the signal beam. Both two beams pass through a combination of cylindrical lens (with about focal distance to the microscope lens) and a 20× microscope objective (NA = 0.65). In this way an elliptical spot (with dimensions 3.5 μm × 180 μm, FWHM) is generated on the input facet, thus generating a well collimated beam inside the sample with negligible diffraction along the transverse z -direction. The two collimated light beams intersect inside the planar waveguide under an angle 2 θ , and are out-coupled by a 10× microscope objective (NA = 0.3). Two photo-detectors are used to periodically measure the output light powers of the two beams with the help of a light chopper. 3 Results and discussion Fig. 2 a and b depicts TE and TM mode spectra at a wavelength of 632.8 nm, respectively. As one can see in Fig. 2 a, the effective refractive index of the fundamental mode (TE 0 ) is higher than the substrate index n e , whilst, as shown in Fig. 2 b, all effective indices of the observed TM modes are below the ordinary index n o . We reconstructed both n e and n o index profiles based on the reflective calculation method (RCM) [20] (see Fig. 3 ). The average penetration depth of the incident protons is 3.5 μm (calculated by SRIM 2010 code [21] ). As one can see, the n e profile is of typical “well + barrier” shape, which is in good agreement with those of ion-implanted CLN waveguides [16] . On the other hand, the n o profile is of the “barrier” type, which only depends on the negative index barrier at then end of the ion range. In such a case, the in-coupled light is strongly damped due to light tunneling into the substrate. As we are merely interested in the photorefractive properties of the sample, and because the (extraordinarily) TE-polarized light induces larger index changes (due to larger electro-optic coefficients, r 33 ≈ 3 r 31 ), two-wave mixing is performed using extraordinarily polarized modes only. When the refractive index grating generated by two interfering beams is not in phase (or out of phase) with the light intensity pattern, a signal beam (index s ) can be amplified by a (usually much stronger) pump beam (index p ) by two-wave mixing (TWM). In LiNbO 3 , a grating component shifted by π /2 is induced by the diffusion mechanism of charge transport, while the domination photovoltaic mechanism generates an out-of-phase index change only [4,6] . The beam power ratio β 0 and TWM gain factor γ 0 are defined as follows: (1) β 0 = P s P p , (2) γ 0 = I s ( with pump ) I s ( without pump ) , where P s and P p are the powers of signal and pump beam, respectively. Here I s (without pump) is the intensity of the transmitted signal when the pump beam is off, while I s (with pump) is signal intensity for pump beam on and when the grating has built up until saturation. We obtained the values of P s and P p inside the sample by measuring the out-coupled power, corrected by Fresnel reflection losses of the crystal of ∼14%. A coupling efficiency of ∼70% has been assumed, which accounts for defects on the crystal’s end facet as well as for the imperfect modal field match of incident light and waveguide mode. The accuracy of the power values inside the sample is estimated to be about 20%, while power ratios are assumed to be accurate within 10%. Fig. 4 shows the dependence of the TWM gain factor γ 0 on the (full internal) intersection angle 2 θ , when the incident beam power ratio of signal and pump beam is set to β 0 = 0.02. As one can see, for the investigated range the variation of the TWM gain factor γ 0 with intersection angle is rather small. In fact, in this range of 2 θ the diffusion space-charge field responsible for beam coupling typically reaches a plateau-like maximum [22] . For simplicity, in the present work, the intersection angle 2 θ has been fixed to be 11°. In this case, the period of the corresponding photorefractive grating is Λ = 1.5 μm. Diffraction efficiency η was measured at the same time with β 0 = 0.02, and a maximum value of η = 20% is obtained for an angle of 2 θ = 11°. According to Kogelnik’s equation (3) η = I diffracted I diffracted + I transmitted = sin 2 π Δ nL m λ cos ( θ ) , with L being the (effective) thickness of the sample, m is the modulation ratio and θ is the half coupling angle, we find Δ n = 1.2 × 10 −5 . This (saturated) refractive index change Δ n decreases only very slightly when the coupling angle is changed. In Fig. 5 the TWM gain factor γ 0 as a function of incident beam power ratio β 0 is monitored. To calculate the TWM gain coefficient Γ , we perform a numerical simulation of the experimental data, which was first raised by Fluck et al. [23] for two-dimensional analysis of (focused) Gaussian beams. When the full intersection angle 2 θ of the beams inside the waveguide is 11° (effective interaction length of the two beams is L eff ≈ 1.9 mm in this case), the best fit to the experimental data results in an exponential gain coefficient of Γ = 15 cm −1 . The measured TWM response time τ vs. total incident power P is depicted in Fig. 6 . When the power of the pump light is 1 μW, the response time is about 170 s. The value of τ decreases significantly when the pump power P increases; however, it seems that for P > 6 μW the change of τ is not obvious. In this work, we obtain the lowest τ value of 5 s at P = 13 μW. Fig. 7 shows the measured inverse response time τ vs. total incident intensity I . Here we calculate intensity I as power P divided by the cross section S of the waveguide. For our sample we find S = (3.5 μm) × (180 μm) = 6.3 × 10 −4 mm 2 , obtained from the waveguide’s index profile and the measured FWHM of lateral Gaussian intensity profile of the beam, respectively. The inverse of the (Maxwell) time constant τ is proportional to the product of specific photoconductivity σ ph ,0 and intensity I : (4) τ - 1 = σ ph , 0 ε 0 ε 33 I . Here ε 0 is dielectric constant in vacuum and ε 33 = 32 [7] is the static dielectric constant for SLN. We find a linear fit to the complete set of experimental data, which yields the specific photoconductivity σ ph ,0 = 1 × 10 −15 mV −2 . In a similar work, photorefractive properties of optical waveguides fabricated in Fe:CLN crystals using O 3+ ion implantation were investigated [24] . In such samples a quite similar photorefractive performance was found: The gain coefficients Γ of Fe:SLN and Fe:CLN are 15 cm −1 and 18 cm −1 , respectively, with a shorter response time obtained for Fe:SLN. Furthermore, the photoconductivity σ ph ,0 calculated according to Eq. (3) for Fe:SLN is found to be about one order of magnitude larger when compared to the one in Fe:CLN. 4 Summary We have demonstrated, for the first time, the photorefractive properties of H + -ion implanted Fe-doped near-stoichiometric lithium niobate waveguides by investigating two-wave mixing at a wavelength of 632.8 nm. The maximum wave mixing gain coefficient is 15 cm −1 . For a very low optical input power of only 13 μW the response time of the grating build-up is as low as 5 s, which is significantly shorter than for bulk crystals of the same composition. Acknowledgments This work has been supported by the Program for New Century Excellent Talents in University of China (No. NCET-08-0331), National Science Fund for Distinguished Young Scholars of China (No. 10925524) and the National Basic Research Program of China (973 Program, No. 2010CB832906). References [1] J.M. Dudley G. Genty S. Coen Rev. Mod. Phys. 78 2008 1135 1184 [2] R.L. Espinola J.I. Dadap R.M. Osgood S.J. McNab Y.A. Vlasov Opt. Express 13 2005 4341 4349 [3] Y. Guo Y. Liao L. Cao G. Liu Q. He G. Jin Opt. Express 12 2004 5556 5561 [4] D. Kip Appl. Phys. B 67 1998 131 150 [5] K.K. Wong Properties of Lithium Niobate 2002 INSPEC London [6] K. Buse Appl. Phys. B 64 1997 391 407 [7] L. Arizmendi Phys. Status Solidi A 201 2004 253 283 [8] J. Kang M. Lee S. Lee K. Lim K. Somu S. Takekawa K. Kitamura Appl. Phys. Lett. 85 2004 4367 4369 [9] K. Buse, J. Imbrock, E. Krätzig, K. Peithmann, Photorefractive effects in LiNbO 3 and LiTaO 3 , in: P. Günter, J.-P. Huignard, (Eds.), Photorefractive Materials and their Applications 2: Materials, Springer, New York, 2007. [10] D. Jaque E. Cantelar G. Lifante Appl. Phys. B 88 2007 201 204 [11] E.M. Rodríguez D. Jaque E. Cantelar F. Cussó G. Lifante A.C. Busacca A. Cino S.R. Sanseverino Opt. Express 15 2007 8805 8811 [12] P.D. Townsend P.J. Chandler L. Zhang Optical Effects of Ion Implantation 1994 Cambridge Univ. Press Cambridge, UK [13] F. Chen X.L. Wang K.M. Wang Opt. Mater. 29 2007 1523 1542 [14] F. Chen J. Appl. Phys. 106 2009 081101 [15] F. Chen Y. Tan A. Ródenas Opt. Express 16 2008 1620 16209 16214 [16] L. Wang Q.-M. Lu Appl. Opt. 48 2009 2619 2624 [17] P.F. Borbui R.G. Norwood D.H. Jundt M.M. Fejer J. Appl. Phys. 71 1992 875 879 [18] E.E. Robertson R.W. Eason C. Kaczmarek P.J. Chandler X.F. Huang Opt. Lett. 21 1996 641 643 [19] P.K. Tien R. Ulrich J.R. Martin Appl. Phys. Lett. 14 1969 291 293 [20] P.J. Chandler F.L. Lama Opt. Acta 33 1986 127 142 [21] J.F. Ziegler, Computer Code, SRIM < http://www.srim.org >. [22] N. Kukhtarev V. Markov S. Odoulov M. Soskin V. Vinetskii Ferroelectrics 22 1979 946 960 [23] D. Fluck S. Brülisauer P. Günter Opt. Commun. 115 1995 626 636 [24] Y. Tan F. Chen D. Kip Appl. Phys. B 94 2009 467 471
更多查看译文
关键词
Ion implantation,Stoichiometric LiNbO3,Optical waveguides,Two-wave mixing
AI 理解论文
溯源树
样例
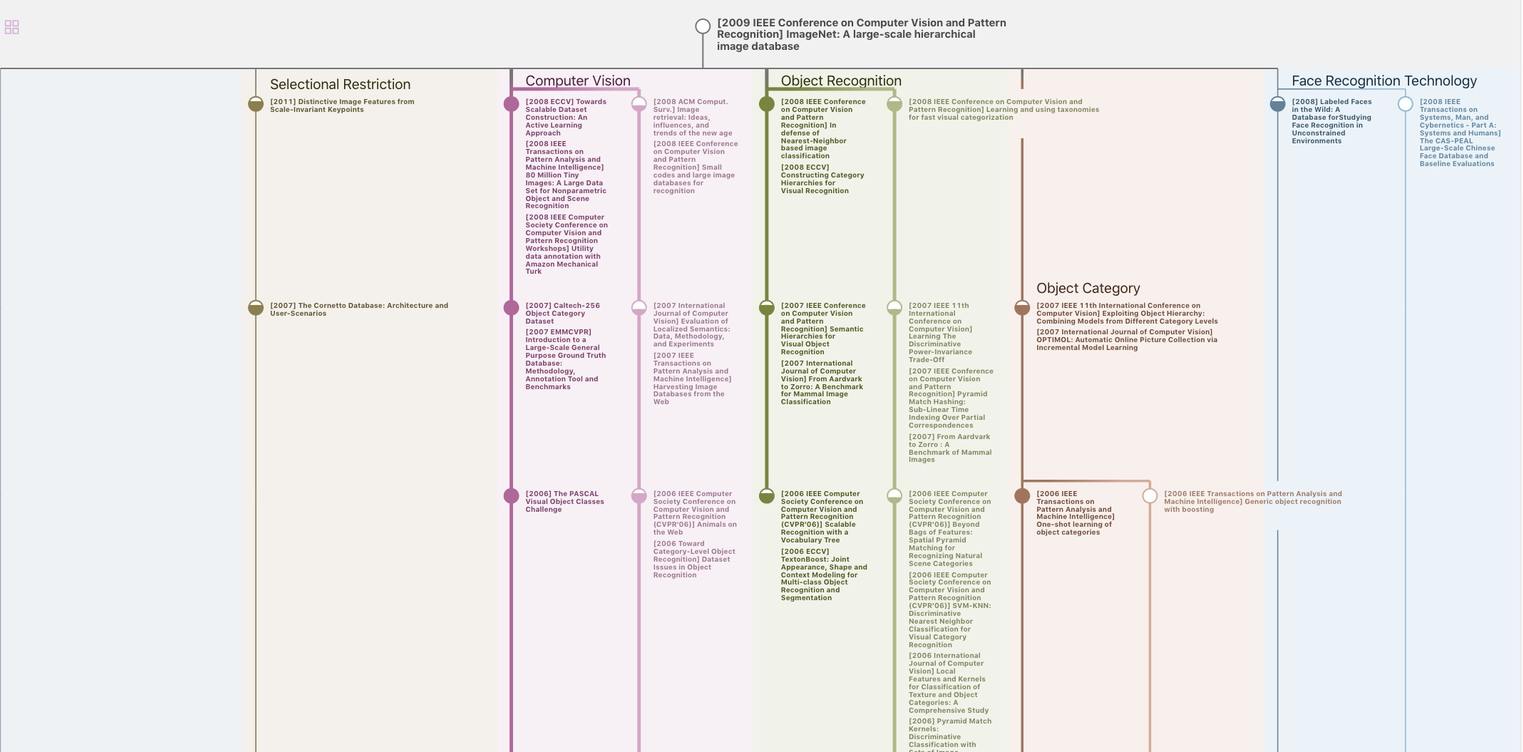
生成溯源树,研究论文发展脉络
Chat Paper
正在生成论文摘要